the Creative Commons Attribution 4.0 License.
the Creative Commons Attribution 4.0 License.
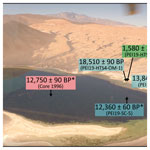
Spatial variability of the modern radiocarbon reservoir effect in the high-altitude lake Laguna del Peinado (southern Puna Plateau, Argentina)
Paula A. Vignoni
Francisco E. Córdoba
Rik Tjallingii
Carla Santamans
Liliana C. Lupo
Achim Brauer
The high-altitude lakes of the Altiplano–Puna Plateau in the Central Andes commonly have large radiocarbon reservoir effects. This, combined with the general scarcity of terrestrial organic matter, makes obtaining a reliable and accurate chronological model based on radiocarbon ages a challenge. As a result, age–depth models based on radiocarbon dating are often constructed by correcting for the modern reservoir effect, but commonly without consideration of spatial and possible temporal variations of reservoir ages within the lake and across the basin. In order to get a better constraint on the spatial variability of the radiocarbon reservoir effects, we analyse 14C ages of modern terrestrial and aquatic plants from the El Peinado basin in the southern Puna Plateau, which hosts Laguna del Peinado fed by hydrothermal springs. The oldest 14C ages of modern samples (> 18 000 and > 26 000 BP) were found in hot springs discharging into the lake, likely resulting from the input of 14C-depleted carbon from old groundwater and 14C-free magmatic CO2. In the littoral and central part of Laguna del Peinado, 14C ages of modern samples were several thousand years younger (> 13 000 and > 12 000 BP) compared to the inflowing waters as a result of CO2 exchange with the atmosphere. Altogether, our findings reveal a spatial variability of up to 14 000 14C years of the modern reservoir effect between the hot springs and the northern part of the Peinado lake basin. Temporal changes of reservoir effects in sediment records are more difficult to quantify, but 14C ages from a short core from Laguna del Peinado may suggest temporal reservoir age variations of a few thousand years. This study has implications for accurate 14C-based chronologies for palaeoclimate studies in the Altiplano–Puna Plateau and similar settings. Our results highlight the need to consider spatial and likely also temporal variations in the reservoir effects when constructing age–depth models.
- Article
(4766 KB) - Full-text XML
- BibTeX
- EndNote
The Altiplano–Puna Plateau is the second highest and largest plateau in the world after Tibet (Allmendinger et al., 1997). It extends along the Central Andes from southern Peru to northern Argentina and Chile at an altitude above 3000 m a.s.l. and is characterised by endorheic basins that host numerous saline lakes, playa lakes, and salars. These systems are highly sensitive to small fluctuations in the water balance, making them promising sensors for studying recent and past environmental and hydrological changes (e.g. Grosjean et al., 1997; Valero-Garcés et al., 2000; McGlue et al., 2013; Santamans et al., 2021) associated with the dynamics of the South American monsoon system (SAMS) and the more northerly influence of the Southern Hemisphere Pacific westerlies (SHPW; Vuille and Ammann, 1997; Garreaud, 2009; Vuille et al., 2012).
Our understanding of the regional and temporal hydroclimatic dynamics in the Altiplano–Puna Plateau is hampered by the difficulty in obtaining accurate chronologies from lacustrine sediments due to the scarcity of terrestrial organic matter and the anomalously old apparent 14C age of waters and hence aquatic samples, known as the “reservoir effect” (Grosjean et al., 1995, 1997, 2001; Geyh et al., 1998; Valero-Garcés et al., 2000; Yu et al., 2007). In particular, in the southern sector of the Andean Plateau (i.e. the Puna region; Fig. 1), no other palaeoclimatic archives (e.g. ice glaciers) exist that can be studied and used to develop high-resolution palaeoclimatic and palaeoenvironmental reconstructions like those located further north in the Altiplano (e.g. Thompson et al., 1995, 1998, 2000; Zech et al., 2008). Therefore, obtaining reliable chronological models using lake sediments from this region is critical and requires an understanding of the 14C reservoir effect variability in each particular lake system. Reservoir effects depend on different causes including CO2 exchange rates between the water and the atmosphere, the internal system mixing dynamics, and the input of 14C-free (“dead”) or 14C-depleted carbon, derived from either dissolved carbonates, volcanic CO2, or the inflow of old groundwater (Macdonald et al., 1991; Ascough et al., 2010; Keaveney and Reimer, 2012; Jull et al., 2013; Lockot et al., 2015). Since the application of other dating methods is also strongly limited or not possible (like 210Pb and 137Cs due to the low concentration of these isotopes at these latitudes and altitudes; Argollo et al., 1994; Cisternas and Araneda, 2001), the construction of chronological models based on corrections for the modern reservoir effect is a common practice (e.g. Grosjean et al., 1995, 1997, 2001; Geyh et al., 1998, 1999; Moreno et al., 2007; Giralt et al., 2008; Jara et al., 2019). Sometimes, even assumptions on temporal variations of the reservoir effect are included in the construction of age–depth models (e.g. Grosjean et al., 2001; Moreno et al., 2007). In contrast, spatial variations within a lake system are less considered, although these can be equally crucial for reliable age–depth models.
Therefore, the aim of this study is to present and discuss new radiocarbon data from various types of modern samples from a range of different locations within the Laguna del Peinado lake system and catchment. This high-altitude lake in the southern Puna Plateau is fed by hydrothermal springs, and previous studies assumed a modern reservoir effect > 12 000 years for a lake sediment record (Valero-Garcés et al., 1999, 2000). We analysed the 14C ages of live/modern terrestrial and aquatic plants within the El Peinado basin to investigate whether significant variability exists and if it follows a spatial trend, as well as the likely sources of the reservoir effect. Furthermore, we report down-core 14C ages from a lake core and compare them with the previously published dates to estimate possible temporal variability of the reservoir effect in the sedimentary record of this lake.
Laguna del Peinado is a shallow lake located at 3760 m a.s.l. in the southern Puna Plateau in the Central Volcanic Zone of the Andes of Argentina (26∘30′16.87′′ S, 68∘5′49.95′′ W; Fig. 1). The lake is located in a topographically closed basin along the Peinado lineament, a NNE–SSW dextral transcurrent fault system that runs along the Antofalla salar axis and presumably continues under the Peinado composite volcano (Seggiaro et al., 2006; Grosse et al., 2022). Peinado is a young, potentially active volcano (last activity dated to 36.8 ± 3.8 ka; 5,890 m a.s.l.) located ∼ 5 km south of Laguna del Peinado (Grosse et al., 2022). It consists of a very steep cone surrounded by a ring of lava originating from several lateral vents, loose scoria, and subordinate pyroclastic flows of dominantly mafic compositions (basaltic andesites and andesites; Grosse et al., 2014, 2017, 2022). The Peinado volcanic field also hosts 17 mafic monogenetic centres, with 7 of them located between the Antofalla salar and the Peinado stratovolcano with Pleistocene ages (0.6 ± 0.1 to 0.15 ± 0.02 Ma; Grosse et al., 2020). Lava from one of these monogenetic centres (0.38 ± 0.02 Ma; Grosse et al., 2020) limits the southern part of the Laguna del Peinado system (Fig. 1). To the west of the lake, the Pliocene rhyolitic Laguna Amarga ignimbrites (3.7–4 Ma, > 70 km3) originated from the Laguna Amarga caldera crop-out (Kay et al., 2010 and references therein; Fig. 1). Four lacustrine terraces have been described, the uppermost consisting of volcanic sandstones and conglomerates cemented with calcite and the lower ones composed of intraclastic and biomicritic limestones (Valero-Garcés et al., 2001).
The main water body in the El Peinado basin is Laguna del Peinado with a maximum length in the N–S direction of 3.4 km, a width of 1.2 km, and a maximum water depth of ∼ 4 m (Fig. 1). The lake is fed by hydrothermal springs located on the southern and western shores. The southern part of the lake is characterised by an extensive shallow wetland area where hot spring seeps and a pool discharge through a stream into the lake, while on the western shore, several smaller hot spring seeps occur. The smaller and shallower Laguna Turquesa, which is currently disconnected due to the low water level (Fig. 1), is located to the north of Laguna del Peinado. Both lakes were connected until ca. 2005 according to satellite images (Villafañe et al., 2021). Carbonate precipitation takes place within both lakes and the hydrothermal spring environments as a result of CO2 degassing, evaporation, and biological processes with deposits comprising a wide variety of facies including microbialites (travertines, microbial mounds, microbial mats, and others) and fine-grained mineral precipitates (Valero-Garcés et al., 2001; Farías et al., 2020; Della Vedova et al., 2023; Vignoni et al., 2022).
The location of the El Peinado basin in the southern portion of the Puna Plateau covers the climatic transition zone between the South American monsoon system (SAMS) and the more northerly influence of the Southern Hemisphere Pacific westerlies (SHPW; Fig. 1). Due to the meridional extension and prominent orography, the Central Andes act as a topographic barrier to the moisture-bearing easterly winds, resulting in a steep E–W rainfall gradient with high precipitation on the eastern flanks and increasing aridity westwards into the Puna Plateau (Strecker et al., 2007; Garreaud, 2009; Castino et al., 2017). In the study region, mean annual precipitation values are < 120 mm yr−1, whereas evaporation has been estimated to be > 1,500 mm yr−1 in Laguna del Negro Francisco located ∼ 150 km southeast of Laguna del Peinado (Grosjean et al., 1997; Strecker et al., 2007). The scarce precipitation events that reach these dry and high-elevation zones are associated with seasonal changes in the position and intensity of the two dominant atmospheric circulation systems (SAMS vs. SHPW; Fig. 1). Approximately 80 % of the annual precipitation occurs during summer when the strengthening of the SAMS and the heating effect over the Puna Plateau are responsible for convective rainfall events (Garreaud, 2009; Vuille et al., 2012). During winter, precipitation is associated with northward incursions of the SHPW, and snowfall events result from Pacific cold fronts often combined with blocking episodes in the South Pacific and polar air isolated cells that migrate north, interacting with warmer tropical air masses (Vuille and Ammann, 1997).
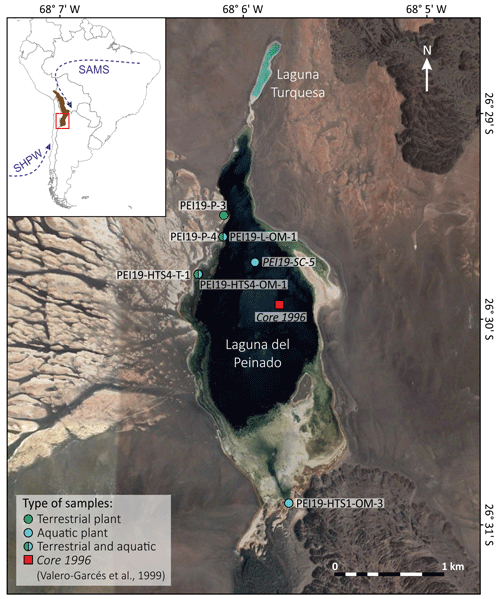
Figure 1Location and type of samples collected in the El Peinado basin during 2019 (© Google Earth 2020, Maxar Technologies, CNES/Airbus). Sediment core samples are indicated in italics. Left top corner: map of South America with the Altiplano–Puna Plateau highlighted in brown and the climatic moisture sources (SAMS: South American monsoon system, SHPW: Southern Hemisphere Pacific westerlies). The red square marks the approximate location of the El Peinado basin in the Puna Plateau of NW Argentina.
3.1 Fieldwork and sampling
During fieldwork in January and November 2019, sediment and organic matter samples including living/modern terrestrial and aquatic plants were collected for radiocarbon analysis from different parts of the lake basin (Fig. 1). Short sediment cores (< 1 m) were retrieved from Laguna del Peinado in two profiles at different water depths using a Uwitec coring device. The cores were split into two halves, photographed, and described at the German Research Centre for Geosciences (GFZ) in Potsdam (Germany). Four bulk samples were sieved for organic macro-remains used for radiocarbon dating from a short core in the central part of the basin at 3.2 m water depth (PEI19-SC-5, Fig. 1). In total, we collected 10 samples: 7 modern samples (3 terrestrial plants, 2 microbial mats from the hot springs, aquatic macrophytes from the lake littoral zone, and aquatic macrophytes from the sediment core-top at 0–2 cm depth) and 3 plant macrofossil samples (from the sediment core at 22–23, 48–49, and 71–72 cm depth). All samples were washed with demineralised water and dried in the oven at 60 ∘C. Plant materials are reported in Table 1, and modern samples are shown in Fig. 2.
3.2 Analysis of radiocarbon and carbon isotopes
Sample preparation, chemical pretreatments, and accelerator mass spectrometry (AMS) 14C measurements were carried out in the Poznan Radiocarbon Laboratory (Poland). A full description of the procedures can be accessed at https://radiocarbon.pl/en/sample-preparation/, last access: 21 May 2023. After mechanical removal of macroscopic contamination under binoculars, the samples underwent a sequential acid–base–acid (ABA) treatment following the protocols established for each material (UW protocol for the wood sample PEI19-P-3 and UV protocol for all other plant samples). Samples were first treated with 1 M HCl at 80 ∘C for 20 min or longer if needed until gas bubble emanations finished (UV, UW), followed by 0.1 M NaOH treatment at room temperature for fragile plant remains (UV) and 80 ∘C for wood (UW), and then 0.25 M HCl at 80 ∘C for 1 hr. After each treatment, samples were rinsed with deionised water (Millipore) to pH = 7. The NaOH treatment step is repeated a few times until no more colouring of the solution caused by humic acids is observed. For the wood sample PEI19-P-3 (UW), an additional treatment with 5 % NaClO2 at room temperature was applied for 30 min. The resulting 14C ages are listed in Table 1 with 1 standard deviation (σ). Samples with percentage of modern carbon (pMC) and radiocarbon ages were converted to fraction modern 14C (F14C) values (Stuiver and Polach, 1977; Reimer et al., 2004; Stenström et al., 2011) using the R package “rintcal” (Blaauw, 2023). Two post-bomb dates from terrestrial plant samples collected at the shore without contact to lake water were calibrated with CALIBomb (Reimer et al., 2004; Reimer and Reimer, 2023) using the Southern Hemisphere Zone 1–2 calibration data set (Hua et al., 2022).
We conducted a simple end-member mixing model to calculate the approximate proportion of dead (14C-free) versus modern (atmospheric) carbon in each sample following Pasquier-Cardin et al. (1999) as
We considered sample PEI19-P-4 as the reference plant that best represents local atmospheric F14C (Table 1) at the time of sampling (2019) compared to the average value for Southern Hemisphere Zone 1–2 (1.019; Hua et al., 2022). We assumed that the 14C content in this sample was in equilibrium with the local atmospheric carbon.
Additionally, δ13Ccarb was analysed in four samples from the carbonatic matrix sediments at 0–2, 24–26, 46–48, and 71–72 cm depths from the core where the plant macrofossils have been taken; δ13Ccarb was analysed in one sample from the microbial mats in the southern hot spring. Samples were frozen for 24 to 48 h, freeze-dried for 72 h, and ground to powder. Carbon isotope analysis of carbonate powders (δ13Ccarb) was carried out on an automated carbonate extraction device (KIEL IV) coupled to a Finnigan MAT 253 IRMS (Thermo Fisher Scientific) at the GFZ in Potsdam. In brief, acid digestion of carbonates with phosphoric acid takes place in the KIEL IV to produce CO2 that is ultimately analysed for δ13Ccarb in the coupled MAT 253 IRMS. Results are expressed in the conventional δ notation in per mille (‰) relative to VPDB (Vienna Pee Dee Belemnite; Table 1). Repeated measurements of the reference material NBS 19 ensured an analytical precision better than ± 0.07 ‰ (σ).
Radiocarbon dating results of modern terrestrial and aquatic plants showed a wide variety of ages in the lake and catchment area (Table 1). Only two samples of terrestrial plants located approximately 15 and 5 m away from the lake shore gave modern ages, PEI19-P-3 (Adesmia sp., 112.39 ± 0.31 pMC) dated to 1994–1996 cal CE and PEI19-P-4 (Poaceae, 101.61 ± 0.34 pMC) to 2018–2019 cal CE (Figs. 1, 2a, and b). Another terrestrial plant (Poaceae) collected near a hot spring (∼ 15 cm) on the western shore (Figs. 1 and 2d) resulted in an age of 1580 ± 30 BP. In contrast, a microbial mat sample of this hot spring was dated to 18 510 ± 90 BP (Figs. 1 and 2c). The oldest measured age was found in microbial mats from the southern shore hot spring pool (26 500 ± 1300 BP; Figs. 1 and 2e). Living aquatic macrophytes (Potamogetonaceae) on the northwestern lake shore (∼ 20 cm water depth) and from the surface sediment taken from the core located ∼ 370 m away (3.2 m water depth) showed similar ages of 13 840 ± 70 and 12 360 ± 60 BP, respectively (Figs. 1, 2f and g). The three down-core samples from aquatic macrophytes (Potamogetonaceae) are in chronostratigraphic order and revealed ages of 16 180 ± 80 BP (22 to 23 cm), 16 720 ± 90 BP (48 to 49 cm), and 17 680 ± 90 BP for the basal layer (71 to 72 cm; Fig. 3).
A simple end-member mixing model revealed the highest proportion of dead carbon in modern microbial mats from the southern and western hot springs (96.4 % and 90.2 %, respectively), while values for the lake modern aquatic macrophytes ranged between ∼ 78 % and 82 % (Table 1, Fig. 2).
Table 114C ages from El Peinado basin. F14C values were calculated with the package “rintcal” (Blaauw, 2023). The proportion of dead (14C-free) carbon was calculated with reference to sample PEI19-P-4, considered representative of the local atmospheric F14C. As a reference, for the year 2019 when these samples were collected, the mean value of atmospheric F14C for the Southern Hemisphere Zone 1–2 is 1.019 (January to May; Hua et al., 2022). The δ13C values in italics correspond to samples at 24 to 26 cm and 46 to 48 cm, and they differ at the sampling depths for 14C. Question marks (?) denote samples where water influence, water mixing, and plant genus and/or species could not be determined with certainty.
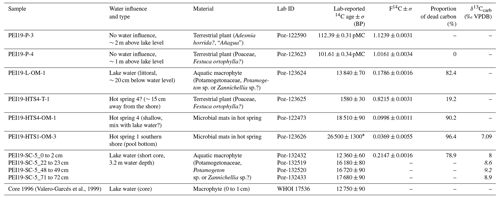
* The high σ is due to the small sample size (0.05 mgC).
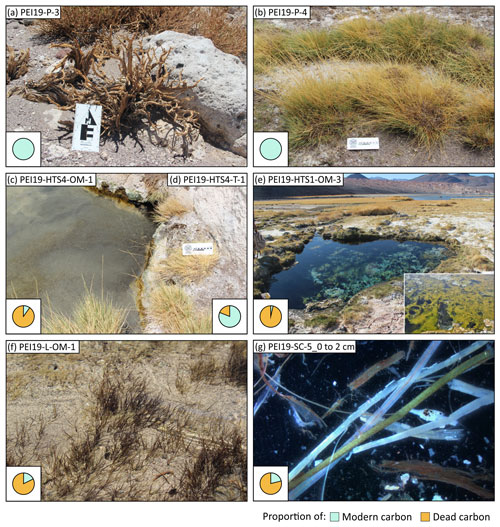
Figure 2Modern samples: (a, b) terrestrial, (c) aquatic, and (d) terrestrial by the western shore hydrothermal spring; (e) aquatic from the southern shore hydrothermal spring; (f) lake littoral and (g) aquatic from the top of the lake short core. The pie charts in the bottom corners show the estimated proportion of modern and dead carbon for each sample (Table 1).
In this section, we discuss the variability observed in the 14C ages of modern plants in the El Peinado basin and the possible sources of 14C-free or 14C-depleted carbon causing reservoir effects that may also be of relevance for other basins in the Altiplano–Puna Plateau as well as basins in similar settings.
5.1 Spatial variability of the 14C reservoir effect in the El Peinado basin
Present-day terrestrial plants are commonly expected to provide modern radiocarbon ages, while aquatic plants potentially take up old carbon. The only two terrestrial plant samples in our study that yielded recent ages and were free of any reservoir effects were found 15 and 5 m away from the lake shore line (Figs. 1 and 4). PEI19-P-3, a woody plant of the genus Adesmia, was most likely dead at the time of sampling as it had no sprouts (Fig. 2a, the front plant was sampled), explaining the high F14C value of 1.1239 ± 0.0031 (Table 1), which corresponds to the atmospheric F14C of year 1994–1996 CE (Hua et al., 2022). PEI19-P-4, a Poaceae, possibly Festuca ortophylla, is dated to 2018–2019 cal CE in agreement with the sampling year (Table 1, Fig. 2b). Another Poaceae sample (PEI19-HTS4-T-1) growing in the vicinity of a hydrothermal spring (∼ 15 cm) revealed an age of 1580 ± 30 BP, indicating incorporation of 14C-depleted carbon (∼ 20 %; Table 1, Fig. 2d). The ages of all modern aquatic samples varied substantially between 12 360 ± 60 and 26 500 ± 1300 BP, showing distinct reservoir effects depending on the location within the basin. The oldest age was found for the modern microbial mats from the southern shore hydrothermal pool, whereas microbial mats from a hot spring at the western shore displayed an age approximately 8000 14C years younger (Table 1, Figs. 1 and 4). Ages from aquatic plants from a nearshore site and surface sediment from the central part of the lake reveal significantly younger ages than those from microbial mats in both hot springs (Table 1, Figs. 1 and 4). From those two, the shallow water sample is ca. 1500 years older (13 840 ± 70 BP) than the sample from the central part of the lake (12 360 ± 60 BP). The general trend of decreasing ages from the inflowing hydrothermal waters and littoral positions towards the deeper lake likely reflects longer residence time of water in the lake and prolonged exchange of the dissolved inorganic carbon (DIC) with the atmospheric CO2 compared to the hydrothermal inflows, resulting in higher 14C concentrations and consequently lower reservoir effects (Table 1, Figs. 1 and 4). In contrast, the higher reservoir effect recorded in the plants from the hot springs and the littoral zone of the lake reflects poorly equilibrated DIC. The difference of ca. 8000 14C years between the two hot springs could result from either some influence (i.e. mixing) of lake water with higher 14C concentrations in the western shore hot spring or the existence of separate hydrothermal systems bounded by the Peinado lineament with distinct 14C content in the DIC (Table 1, Figs. 1 and 4). A similar pattern of spatial variability has been observed in lacustrine systems in the Tibetan Plateau, with high reservoir effect in tributaries and spring waters and a lower reservoir effect in the central regions of lakes with differences of up to 19 000 14C years between different locations within individual systems (Mischke et al., 2013).
5.2 14C reservoir effect sources in the El Peinado basin
5.2.1 Catchment geology
The dissolution of carbonate-rich sediments or rocks in the catchment area is usually considered a main source of 14C-free carbon influx into a lake (Macdonald et al., 1991; Ascough et al., 2010). However, the dissolution of catchment carbonates can only be a minor source of 14C-free carbon into Laguna del Peinado because the lithology of the basin is dominated by volcanic rocks (Fig. 1; Seggiaro et al., 2006; Grosse et al., 2020, 2022) and extreme arid conditions prevail. Carbonatic outcrops are scarce and limited to a few ancient lake terraces consisting of conglomerates and sandstones with volcanic clasts cemented/coated with calcite, intraclastic and biomicritic limestones, and microbialites (Valero-Garcés et al., 2001; Villafañe et al., 2021). Furthermore, calcium available for carbonate formation in this lacustrine system is interpreted as being derived from the alteration of the volcanic bedrock by fluids at high temperatures as described in other lake systems in the Altiplano–Puna Plateau (e.g. Laguna Pastos Grandes; Muller et al., 2020).
5.2.2 Groundwater effects
Another common source of 14C-depleted carbon in lakes can be the contribution of old groundwater (Macdonald et al., 1991; Riggs, 1984; Godfrey et al., 2021). In the Altiplano–Puna Plateau, the contribution of old groundwater to lacustrine systems might be substantial, given the present-day negative water balance regime (Grosjean et al., 1995). Furthermore, water tables can be very deep and develop groundwater flow paths with long transit times that often cross topographic boundaries prior to emerging at the basin bottoms (Moran et al., 2021). The old 14C ages of modern aquatic organisms in the hot springs (Table 1, Fig. 4) can be partially explained by an old origin and high residence times of water in the hydrothermal groundwater system. This is supported by geochemical studies suggesting a meteoric origin of water in the hydrothermal reservoir (Vignoni et al., 2022), which must have occurred in the past given the extremely low present-day precipitation. Groundwater in this region is thought to have formed during wet periods at the end of the last glaciation and early Holocene like the widespread Central Andean Pluvial Events (17.5–14.2 and 13.8–9.7 ka; Latorre et al., 2006; Placzek et al., 2009; Gayo et al., 2012), which overlap with the ages obtained for modern samples from the northwestern shore and the top of the lake core. Water from these wet periods, however, would be too young to explain the two older dates of 18 510 ± 90 and 26 500 ± 1300 BP obtained from organisms directly in the hot springs (Table 1, Fig. 4). Therefore, additional sources of 14C-free or 14C-depleted carbon must be involved (see Sect. 5.2.3).
The influence of old groundwater in the lake is consistent with 3H analysis in wetland systems in the southern Puna Plateau, proving that these environments are mainly sustained by old waters that can be centuries to several millennia old, with only a minor contribution of modern water (< 60 years old) not exceeding 10 % (Moran et al., 2019, 2021; Frau et al., 2021). For example, the zero 3H activity in the lake waters of the Carachi Pampa basin (∼ 50 km east of the El Peinado basin) indicates that it is almost entirely sustained by old groundwater (Frau et al., 2021). Also, geochemical (3H, δ18O, δ2H) and hydrophysical studies in springs and groundwater feeding the Salar de Atacama basin, located ∼ 325 km north of the study area, revealed a large regional groundwater system integrated over timescales of 100–10 000 years or longer (Moran et al., 2019). Although 3H data of waters are lacking for the El Peinado basin, this system is most likely almost entirely supported by old groundwater as also reported from other sites in the region (e.g. Frau et al., 2021; Moran et al., 2019, 2021; Godfrey et al., 2021).
5.2.3 14C-free volcanic CO2
Another potential source of the large reservoir effects in the El Peinado basin, in particular for those dates exceeding the ages of the Central Andean Pluvial Events, is a contribution of 14C-free magma-derived CO2 (Macdonald et al., 1991, and references therein; Pasquier-Cardin et al., 1999). In the young volcanic El Peinado basin (Grosse et al., 2020, 2022), magma degassing is a likely source of CO2 into the hydrothermal system as assumed for the Cerro Blanco geothermal system located 40 km to the ESE (Chiodi et al., 2019). Geophysical studies also suggested the existence of magma reservoirs in the crust beneath the El Peinado area (Bianchi et al., 2013; Ward et al., 2017). An influence of volcanic CO2 is further supported by permanent bubbling in the southern shore hot spring pool as well as in the seepage areas on the west coast. Furthermore, strong 13C enrichment in the lake carbonates up to +13 ‰ VPDB has been explained by degassing of volcanic CO2 (Table 1; Valero-Garcés et al., 1999). Considering the scenario of different hydrothermal systems on the south and west lake coasts, a higher contribution of magmatic CO2 in the southern hydrothermal reservoir compared to those on the west would explain the oldest age of 26 500 ± 1300 BP recorded in the basin. For example, 14C ages of the DIC exceeding 20 000 years due to dilution by geogenic DIC sources have also been reported from the Loa and Calama basins located north of the Salar de Atacama (∼ 450 km NNW of the El Peinado basin; Godfrey et al., 2021). Moreover, the aquatic plant with the oldest 14C age has a proportion of modern carbon (∼ 4 %; Table 1, Fig. 2d) supporting the finding that the reservoir ages result from dilution with 14C-free volcanic CO2.
Degassing of magmatic CO2 might have also caused the age of 1580 ± 30 BP for a modern terrestrial plant from the western shore (Table 1, Figs. 1 and 2c). It has been reported that diffuse emanations of magmatic CO2 through soils lead to a substantial 14C depletion in terrestrial plants when 14C-free CO2 is assimilated during photosynthesis (Pasquier-Cardin et al., 1999). This might explain the old age of the terrestrial plant sample since it grew at a distance of only ∼ 15 cm from the local hot spring. Potential uptake of soil DIC through the roots might additionally contribute but only to a very minor degree since it usually represents less than 1 % of the total CO2 fixed by plants (Loczy et al., 1983; Brix, 1990; Enoch and Olesen, 1993; Ford et al., 2007). The other two dated terrestrial plants were not affected by volcanic CO2 contamination, probably because they grew about 5 m and 15 m further away from the lake shore and from potential sources of volcanic CO2 as no hot springs were identified in that area (Table 1; Figs. 2a, b, and 4).
5.3 14C reservoir effect in surface sediments
The core-top age obtained from aquatic macrophytes (Potamogetonaceae) from core PEI19-SC-5 (12 360 ± 60 BP) is ∼ 400 14C years younger compared to that of a core taken in 1996 (Valero-Garcés et al., 1999, 2000; Table 1, Fig. 4). A likely explanation for a larger reservoir effect in the previous core is its location closer to the southern hydrothermal spring. This would be in agreement with our observations in the modern environment revealing decreasing reservoir effects with increasing distance to the hydrothermal springs due to extended DIC equilibration with atmospheric CO2.
However, we cannot fully exclude a decrease in the reservoir effects in the last 23 years since the core from Valero-Garcés et al. (1999) was recovered, probably related to a lake-level lowering of at least 0.6 m and the associated disconnection between Laguna del Peinado and Laguna Turquesa (Villafañe et al., 2021). This has been assumed for other lakes in the Altiplano–Puna Plateau (e.g. Laguna Lejía, Laguna Miscanti, Lago Chungará; Geyh et al., 1998, 1999; Grosjean et al., 2001; Moreno et al., 2007; Giralt et al., 2008). In contrast, studies from other lake environments in NW China report an opposite mechanism, i.e. increasing reservoir effect related to lake-level lowering (Zhou et al., 2020). Due to the lack of systematic studies, the influence of short-term lake-level fluctuations on modern reservoir effects remains elusive. Other potential causes for temporal reservoir effect changes might be fluctuations in geothermal activity (Ascough et al., 2010) or changes in the lake primary productivity (Zhou et al., 2020), though we have no evidence to investigate these in more depth.
5.4 Down-core 14C ages
The four down-core 14C ages from aquatic macrophytes (Potamogetonaceae) in our core PEI19-SC-5 are in chronological order (Table 1, Fig. 3). The three radiocarbon ages between ca. 22 and 72 cm core depth suggest that this 50 cm of sediment comprises ca. 1500 years (Fig. 3). The age of 12 360 ± 60 BP from the core surface suggests that the uppermost 22 cm of the sediment record covers a period of ca. 4000 years. Assuming a largely constant reservoir effect, either the sedimentation rate must have decreased or the sediment record includes a major hiatus. We do not observe lithological indications in the sediment core for a substantial sedimentation rate change or for a hiatus in the record. However, since detection of a hiatus is not always straightforward, we cannot fully exclude this possibility. The only alternative interpretation would be a major decrease of the reservoir effect during the deposition of the uppermost 22 cm of the sediment record. Unfortunately, we do not have robust data to support any of these possibilities; hence the age–depth relation of the core remains a matter of debate. Consequently, the age of the base of the core of 17 680 ± 90 BP (Table 1) might also be questioned. Interestingly, our data largely resemble the radiocarbon dates from the previous core taken in 1996 (Valero-Garces et al., 1999), except the reservoir effect of the sediment surface that is a few hundred years lower, likely due to spatial variations within the lake basin as discussed above. However, the age model of the previous core has been re-interpreted based on three preliminary U Th dates implying a much younger age of ca. 450 BP for the base of the 1996 core (Valero-Garces et al., 2000, 2003). Accepting this age, the radiocarbon reservoir effect must have decreased in the last 5 centuries by about 4000–5000 14C years. Due to the lack of a critical assessment of potential bias of U Th dating in this lake setting (Valero-Garces et al., 2000, 2003), the true age of the Peinado sediments remains unsolved. However, even if the good agreement of the radiocarbon dates in both cores does not necessarily prove the absence of major reservoir effect changes, the postulation of a reservoir effect decrease of several thousand years in the last few centuries should be questioned and re-investigated. Further research is needed, not only in this lake but also along the Altiplano–Puna Plateau to understand how these reservoir effect changes vary across the region.
Radiocarbon dating of modern plants revealed large reservoir effects ranging between > 12 000 and > 26 000 14C years within the El Peinado basin. These reservoir effects result from two dominant processes: the inflow of old groundwater that likely formed during pluvial phases at the end of the last glaciation and an additional contribution of 14C-free magmatic CO2. We could further prove that the reservoir effect shows clear differences within the lake basin depending on the distance to the source of old groundwater inflow and volcanic CO2. This can even influence the dating of sediment cores obtained from different locations in the lake. Through comparison of surface sediments with a previously published sediment record, we found indications of a ca. 400-year difference in the reservoir ages between the two cores. Such spatial variations in the 14C reservoir effect may also occur in other lake records in the Central Andes and elsewhere, and these should also be considered for the construction of age models. In this sense, corrections of 14C chronologies based on a single reservoir age for an entire lake are not reliable and would produce inaccurate chronological models as it can vary from hundreds to thousands of years within a lake, leading to temporally misleading palaeoclimatic interpretations. This problem might be solved by either dating truly terrestrial material like pollen or applying independent dating methods like U Th. Both, however, also have deficiencies so that constructing chronologies in environments such as that of Laguna del Peinado remains a major challenge. Nevertheless, the characterisation of spatial variations in reservoir effects has the potential to better assess the underlying processes influencing radiocarbon ages in a lake, even if it does not fully solve the problem of reservoir effect temporal changes.
All data used in this study are available in Table 1 of the paper.
PAV performed the sample processing, conducted the data analyses, and wrote the article with contributions from all co-authors. AB, FEC, and RT designed the project and organised fieldwork together with PAV and CS. LCL helped to identify the families of the plants sampled. AB, FEC, and RT supervised analyses and article writing.
The contact author has declared that none of the authors has any competing interests.
Publisher’s note: Copernicus Publications remains neutral with regard to jurisdictional claims in published maps and institutional affiliations.
We would especially like to thank Santos Vazquez and Pablo A. Martin for their invaluable help with logistics and fieldwork in this extreme region. We thank the Secretaría de Estado del Ambiente y Desarrollo Sustentable and Dirección Provincial de Biodiversidad from Gobierno de la Provincia de Catamarca (Argentina) for granting us the working permits and Comunidad Coya Atacameña de Antofalla for supervising fieldwork. We would also like to acknowledge Tomasz Goslar, head of the Poznan Radiocarbon Laboratory, for providing us with the necessary information on the sample treatment. We are grateful for the comments and suggestions of the two anonymous reviewers who helped us to improve this paper.
This research has been supported by the Deutsche Forschungsgemeinschaft (grant no. STRATEGy DFG 373/34-1), the Helmholtz-Zentrum Potsdam – Deutsches GeoForschungsZentrum (GFZ), the Agencia Nacional de Promoción Científica y Tecnológica (grant no. PICT-2019-01336), the Consejo Nacional de Investigaciones Científicas y Técnicas (grant no. GII StRATEGy 163-A 1.1), and the Secretaría de Ciencia, Técnica y Estudios Regionales (grant nos. SeCTER-UNJu E/G011 and E/1001/-Integrar).
This paper was edited by Irka Hajdas and reviewed by two anonymous referees.
Allmendinger, R. W., Jordan, T. E., Kay, S. M., and Isacks, B. L.: The evolution of the Altiplano-Puna Plateau of the Central Andes, Annu. Rev. Earth Pl. Sc., 25, 139–174, https://doi.org/10.1146/annurev.earth.25.1.139, 1997.
Argollo, J., Mourguiart, P., Pinglot, J.-F., Pourchet, M., Preiss, N., and Wirmann, D.: Sedimentación reciente en el lago Titicaca (Bolivia), in: Actas Volumen I, 7∘ Congreso Geológico Chileno, Concepción, Chile, 17–21 October 1994, 225–229, 1994.
Ascough, P. L., Cook, G. T., Church, M. J., Dunbar, E., Einarsson, Á., McGovern, T. H., Dugmore, A. J., Perdikaris, S., Hastie, H., Friðriksson, A., and Gestsdóttir, H.: Temporal and Spatial Variations in Freshwater 14C Reservoir Effects: Lake Mývatn, Northern Iceland, Radiocarbon, 52, 1098–1112, https://doi.org/10.1017/S003382220004618X, 2010.
Bianchi, M., Heit, B., Jakovlev, A., Yuan, X., Kay, S. M., Sandvol, E., Alonso, R. N., Coira, B., Brown, L., Kind, R., and Comte, D.: Teleseismic tomography of the southern Puna plateau in Argentina and adjacent regions, 586, 65–83, https://doi.org/10.1016/j.tecto.2012.11.016, 2013.
Blaauw, M.: Package “rintcal”, version 0.5.3, CRAN [package], https://cran.r-project.org/package=rintcal (last access: 21 April 2023), 2023.
Brix, H.: Uptake and photosynthetic utilization of sediment-derived carbon by Phragmites australis (Cav.) Trin. ex Steudel, Aquat. Bot., 38, 377–389, https://doi.org/10.1016/0304-3770(90)90032-G, 1990.
Castino, F., Bookhagen, B., and Strecker, M. R.: Rainfall variability and trends of the past six decades (1950–2014) in the subtropical NW Argentine Andes, Clim. Dynam., 48, 1049–1067, https://doi.org/10.1007/s00382-016-3127-2, 2017.
Chiodi, A., Tassi, F., Báez, W., Filipovich, R., Bustos, E., Glok Galli, M., Suzaño, N., Ahumada, M. F., Viramonte, J. G., Giordano, G., Pecoraino, G., and Vaselli, O.: Preliminary conceptual model of the Cerro Blanco caldera-hosted geothermal system (Southern Puna, Argentina): Inferences from geochemical investigations, J. South Am. Earth Sci., 94, 102213, https://doi.org/10.1016/j.jsames.2019.102213, 2019.
Cisternas, M. and Araneda, A.: Variaciones isotópicas (210Pb, 137Cs) antropogénicas en el registro estratigráfico de un lago de la cordillera de Nahuelbuta, Chile, Rev. Geológica Chile, 28, 105–115, https://doi.org/10.4067/S0716-02082001000100006, 2001.
Della Vedova, M., Villafañe, P. G., Cónsole-Gonella, C., Bahniuk Rumbelsperger, A., Fadel Cury, L., Horta, L. R., and Farías, M. E.: Disentangling microstructure and environmental conditions in high-altitude Andean microbialite systems (Catamarca, Argentine Puna), Environ. Microbiol. Rep., 15, 92–108, https://doi.org/10.1111/1758-2229.13128, 2023.
Enoch, H. Z. and Olesen, J. M.: Plant response to irrigation with water enriched with carbon dioxide, New Phytol., 125, 249–258, https://doi.org/10.1111/j.1469-8137.1993.tb03880.x, 1993.
Farías, M. E., Villafañe, P. G., and Lencina, A. I.: Integral Prospection of Andean Microbial Ecosystem Project, in: Microbial Ecosystems in Central Andes Extreme Environments, edited by: Farías, M. E., Springer, 245–260, https://doi.org/10.1007/978-3-030-36192-1_17, 2020.
Ford, C. R., Wurzburger, N., Hendrick, R. L., and Teskey, R. O.: Soil DIC uptake and fixation in Pinus taeda seedlings and its C contribution to plant tissues and ectomycorrhizal fungi, Tree Physiol., 27, 375–383, https://doi.org/10.1093/treephys/27.3.375, 2007.
Frau, D., Moran, B. J., Arengo, F., Marconi, P., Battauz, Y., Mora, C., Manzo, R., Mayora, G., and Boutt, D. F.: Hydroclimatological Patterns and Limnological Characteristics of Unique Wetland Systems on the Argentine High Andean Plateau, Hydrology, 8, 164, https://doi.org/10.3390/hydrology8040164, 2021.
Garreaud, R. D.: The Andes climate and weather, Adv. Geosci., 22, 3–11, https://doi.org/10.5194/adgeo-22-3-2009, 2009.
Gayo, E. M., Latorre, C., Jordan, T. E., Nester, P. L., Estay, S. A., Ojeda, K. F., and Santoro, C. M.: Late Quaternary hydrological and ecological changes in the hyperarid core of the northern Atacama Desert (∼ 21∘ S), Earth-Sci. Rev., 113, 120–140, https://doi.org/10.1016/j.earscirev.2012.04.003, 2012.
Geyh, M. A., Schotterer, U., and Grosjean, M.: Temporal Changes of the 14C Reservoir Effect in Lakes, Radiocarbon, 40, 921–931, https://doi.org/10.1017/S0033822200018890, 1998.
Geyh, M. A., Grosjean, M., Núñez, L., and Schotterer, U.: Radiocarbon reservoir effect and the timing of the late-glacial/early Holocene humid phase in the Atacama Desert (Northern Chile), Quaternary Res., 52, 143–153, https://doi.org/10.1006/qres.1999.2060, 1999.
Giralt, S., Moreno, A., Bao, R., Sáez, A., Prego, R., Valero-Garcés, B. L., Pueyo, J. J., González-Sampériz, P., and Taberner, C.: A statistical approach to disentangle environmental forcings in a lacustrine record: the Lago Chungará case (Chilean Altiplano), J. Paleolimnol., 40, 195–215, https://doi.org/10.1007/s10933-007-9151-9, 2008.
Godfrey, L. V., Herrera, C., Burr, G. S., Houston, J., Aguirre, I., and Jordan, T. E.: δ13C and 14C activity of groundwater DOC and DIC in the volcanically active and arid Loa Basin of northern Chile, J. Hydrol., 595, 125987, https://doi.org/10.1016/j.jhydrol.2021.125987, 2021.
Grosjean, M., Geyh, M., Messerli, B., and Schotterer, U.: Late-glacial and early Holocene lake sediments, groundwater formation and climate in the Atacama Altiplano 22–24∘ S, J. Paleolimnol., 14, 241–252, 1995.
Grosjean, M., Valero-Garcés, B. L., Geyh, M. A., Messerli, B., Schotterer, U., Schreier, H., and Kelts, K.: Mid- and late-Holocene limnogeology of Laguna del Negro Francisco, northern Chile, and its palaeoclimatic implications, The Holocene, 7, 151–159, https://doi.org/10.1177/095968369700700203, 1997.
Grosjean, M., van Leeuwen, J. F. N., van Der Knaap, W. O., Geyh, M. A., Ammann, B., Tanner, W., Messerli, B., Núñez, L. A., Valero-Garcés, B. L., and Veit, H.: A 22 000 14C year BP sediment and pollen record of climate change from Laguna Miscanti (23∘ S), northern Chile, Glob. Planet. Change, 28, 35–51, https://doi.org/10.1016/S0921-8181(00)00063-1, 2001.
Grosse, P., Orihashi, Y., Guzmán, S., and Petrinovic, I.: Volcanismo cuaternario en la zona del Paso San Francisco, Catamarca, in: Actas XIX Congreso Geológico Argentino, XIX Congreso Geológico Argentino, Córdoba, Argentina, 2–6 June 2014, S24, 2–6, ISBN 978-987-22-4037-0, 2014.
Grosse, P., Guzmán, S., and Petrinovic, I.: Volcanes compuestos cenozoicos del noroeste argentino, in: Ciencias de la Tierra y Recursos Naturales del NOA, Relatorio del XX Congreso Geológico Argentino, edited by: Muruaga, C. M. and Grosse, P., XX Congreso Geológico Argentino, San Miguel de Tucumán, Argentina, 7–11 August 2017, Asociación Geológica Argentina, 484–517, ISBN 978-987-42-6666-8, 2017.
Grosse, P., Ochi Ramacciotti, M. L., Escalante Fochi, F., Guzmán, S., Orihashi, Y., and Sumino, H.: Geomorphology, morphometry, spatial distribution and ages of mafic monogenetic volcanoes of the Peinado and Incahuasi fields, southernmost Central Volcanic Zone of the Andes, J. Volcanol. Geoth. Res., 401, 106966, https://doi.org/10.1016/j.jvolgeores.2020.106966, 2020.
Grosse, P., Guzmán, S. R., Nauret, F., Orihashi, Y., and Sumino, H.: Central vs. lateral growth and evolution of the < 100 ka Peinado composite volcano, southern Central Volcanic Zone of the Andes, J. Volcanol. Geoth. Res., 425, 107532, https://doi.org/10.1016/j.jvolgeores.2022.107532, 2022.
Hua, Q., Turnbull, J. C., Santos, G. M., Rakowski, A. Z., Ancapichún, S., De Pol-Holz, R., Hammer, S., Lehman, S. J., Levin, I., Miller, J. B., Palmer, J. G., and Turney, C. S. M.: Atmospheric radiocarbon for the period 1950–2019, Radiocarbon, 64, 723–745, https://doi.org/10.1017/RDC.2021.95, 2022.
Jara, I., Maldonado, A., González, L., Hernández, A., Sáez, A., Giralt, S., Bao, R., and Valero-Garcés, B.: Centennial-scale precipitation anomalies in the southern Altiplano (18∘ S) suggest an extratropical driver for the South American Summer Monsoon during the late Holocene, Clim. Past, 15, 1845–1859, https://doi.org/10.5194/cp-15-1845-2019, 2019.
Jull, A. J. T., Burr, G. S., and Hodgins, G. W. L.: Radiocarbon dating, reservoir effects, and calibration, Quaternary Int., 299, 64–71, https://doi.org/10.1016/j.quaint.2012.10.028, 2013.
Kay, S. M., Coira, B. L., Caffe, P. J., and Chen, C. H.: Regional chemical diversity, crustal and mantle sources and evolution of central Andean Puna plateau ignimbrites, J. Volcanol. Geoth. Res., 198, 81–111, https://doi.org/10.1016/j.jvolgeores.2010.08.013, 2010.
Keaveney, E. M. and Reimer, P. J.: Understanding the variability in freshwater radiocarbon reservoir offsets: A cautionary tale, J. Archaeol. Sci., 39, 1306–1316, https://doi.org/10.1016/j.jas.2011.12.025, 2012.
Latorre, C., Betancourt, J. L., and Arroyo, M. T. K.: Late Quaternary vegetation and climate history of a perennial river canyon in the Río Salado basin (22∘ S) of Northern Chile, Quaternary Res., 65, 450–466, https://doi.org/10.1016/j.yqres.2006.02.002, 2006.
Lockot, G., Ramisch, A., Wünnemann, B., Hartmann, K., Haberzettl, T., Chen, H., and Diekmann, B.: A Process- and Provenance-Based Attempt to Unravel Inconsistent Radiocarbon Chronologies in Lake Sediments: An Example from Lake Heihai, North Tibetan Plateau (China), Radiocarbon, 57, 1003–1019, https://doi.org/10.2458/azu_rc.57.18221, 2015.
Loczy, S., Carignan, R., and Planas, D.: The role of roots in carbon uptake by the submersed macrophytes Myriophyllum spicatum, Vallisneria americana, and Heteranthera dubia, Hydrobiologia, 98, 3–7, https://doi.org/10.1007/BF00019244, 1983.
Macdonald, A. G. M., Beukens, R. P., and Kieser, W. E.: Radiocarbon Dating of Limnic Sediments: A Comparative Analysis and Discussion, Ecology, 72, 1150–1155, 1991.
McGlue, M. M., Cohen, A. S., Ellis, G. S., and Kowler, A. L.: Late Quaternary stratigraphy, sedimentology and geochemistry of an underfilled lake basin in the Puna plateau (northwest Argentina), Basin Res., 25, 638–658, https://doi.org/10.1111/bre.12025, 2013.
Mischke, S., Weynell, M., Zhang, C., and Wiechert, U.: C reservoir effects in Tibetan Plateau lakes, Quaternary Int., 313/314, 147–155, 2013.
Moran, B. J., Boutt, D. F., and Munk, L. A.: Stable and Radioisotope Systematics Reveal Fossil Water as Fundamental Characteristic of Arid Orogenic-Scale Groundwater Systems, Water Resour. Res., 55, 11295–11315, https://doi.org/10.1029/2019WR026386, 2019.
Moran, B. J., Boutt, D. F., Munk, L. A., and Fisher, J. D.: Pronounced Water Age Partitioning Between Arid Andean Aquifers and Fresh-Saline Lagoon Systems, EGU General Assembly 2021, online, 19–30 Apr 2021, EGU21-13753, https://doi.org/10.5194/egusphere-egu21-13753, 2021.
Moreno, A., Giralt, S., Valero-Garcés, B., Sáez, A., Bao, R., Prego, R., Pueyo, J. J., González-Sampériz, P., and Taberner, C.: A 14 kyr record of the tropical Andes: The Lago Chungará sequence (18∘ S, northern Chilean Altiplano), Quaternary Int., 161, 4–21, https://doi.org/10.1016/j.quaint.2006.10.020, 2007.
Muller, E., Gaucher, E. C., Durlet, C., Moquet, J. S., Moreira, M., Rouchon, V., Louvat, P., Bardoux, G., Noirez, S., Bougeault, C., Vennin, E., Gérard, E., Chavez, M., Virgone, A., and Ader, M.: The origin of continental carbonates in Andean salars: A multi-tracer geochemical approach in Laguna Pastos Grandes (Bolivia), Geochim. Cosmochim. Ac., 279, 220–237, https://doi.org/10.1016/j.gca.2020.03.020, 2020.
Pasquier-Cardin, A., Allard, P., Ferreira, T., Hatte, C., Coutinho, R., Fontugne, M., and Jaudon, M.: Magma-derived CO2 emissions recorded in 14C and 13C content of plants growing in Furnas caldera, Azores, J. Volcanol. Geoth. Res., 92, 195–207, https://doi.org/10.1016/S0377-0273(99)00076-1, 1999.
Placzek, C., Quade, J., Betancourt, J. L., Patchett, P. J., Rech, J. A., Latorre, C., Matmon, A., Holmgren, C., and English, N. B.: Climate in the dry central andes over geologic, millennial, and interannual timescales, Ann. Missouri Bot. Gard., 96, 386–397, https://doi.org/10.3417/2008019, 2009.
Reimer, P. J., Brown, T. A., and Reimer, R. W.: Discussion: Reporting and calibration of post-bomb 14C data, Radiocarbon, 46, 1299–1304, https://doi.org/10.1017/S0033822200033154, 2004.
Reimer, R. W. and Reimer, P. J.: CALIBomb, http://calib.org, last access: 16 January 2023.
Riggs, A. C.: Major Carbon-14 Deficiency in Modern Snail Shells from Southern Nevada Springs, Science, 224, 58–61, https://doi.org/10.1126/science.224.4644.58, 1984.
Santamans, C. D., Cordoba, F. E., Franco, M. G., Vignoni, P., and Lupo, L. C.: Hydro-climatological variability in Lagunas de Vilama System, Argentinean Altiplano-Puna Plateau, Southern Tropical Andes (22∘ S), and its response to large-scale climate forcings, Sci. Total Environ., 767, 144926, https://doi.org/10.1016/j.scitotenv.2020.144926, 2021.
Seggiaro, R., Hongn, F., Folguera, A., and Clavero, J.: Hoja Geológica 2769-II, Paso de San Francisco (Escala 1:250 000), Instituto de Geología y Recursos Minerales, Servicio Geológico Minero de Argentina, Buenos Aires, 294, 1–62, 2006.
Stenström, K. E., Skog, G., Georgiadou, E., Genberg, J., and Johansson, A.: A guide to radiocarbon units and calculations, Internal Report LUNFD6(NFFR-3111)/1-17/(2011), Lund University, Nuclear Physics, Lund, Sweden, 1–17, 2011.
Strecker, M. R., Alonso, R. N., Bookhagen, B., Carrapa, B., Hilley, G. E., Sobel, E. R., and Trauth, M. H.: Tectonics and climate of the southern central Andes, Annu. Rev. Earth Pl. Sc., 35, 747–787, https://doi.org/10.1146/annurev.earth.35.031306.140158, 2007.
Stuiver, M. and Polach, H. A.: Reporting of 14C data, Radiocarbon, 19, 355–363, 1977.
Thompson, L. G., Mosley-Thompson, E., Davis, M. E., Lin, P.-N., Henderson, K. A., Cole-Dai, J., Bolzan, J. F., and Liu, K. -B.: Late Glacial Stage and Holocene Tropical Ice Core Records from Huascarán, Peru, Science, 269, 46–50, https://doi.org/10.1126/science.269.5220.46, 1995.
Thompson, L. G., Davis, M. E., Mosley-Thompson, E., Sowers, T. A., Henderson, K. A., Zagorodnov, V. S., Lin, P. N., Mikhalenko, V. N., Campen, R. K., Bolzan, J. F., Cole-Dai, J., and Francou, B.: A 25,000-year tropical climate history from Bolivian ice cores, Science, 282, 1858–1864, https://doi.org/10.1126/science.282.5395.1858, 1998.
Thompson, L. G., Mosley-thompson, E., and Henderson, K. A.: Ice-core palaeoclimate records in tropical America, J. Quaternary Sci., 15, 377–394, 2000.
Valero-Garcés, B. L., Delgado-Huertas, A., Ratto, N., and Navas, A.: Large 13C enrichment in primary carbonates from Andean Altiplano lakes, northwest Argentina, Earth Planet. Sc. Lett., 171, 253–266, https://doi.org/10.1016/S0012-821X(99)00150-8, 1999.
Valero-Garcés, B. L., Delgado-Huertas, A., Ratto, N., Navas, A., and Edwards, L.: Paleohydrology of Andean saline lakes from sedimentological and isotopic records, Northwestern Argentina, J. Paleolimnol., 24, 343–359, https://doi.org/10.1023/A:1008146122074, 2000.
Valero-Garcés, B. L., Arenas, C., and Delgado-Huertas, A.: Depositional environments of Quaternary lacustrine travertines and stromatolites from high-altitude Andean lakes, Northwestern Argentina, Can. J. Earth Sci., 38, 1263–1283, https://doi.org/10.1139/cjes-38-8-1263, 2001.
Valero-Garcés, B. L., Delgado-Huertas, A., Navas, A., Edwards, L., Schwalb, A., and Ratto, N.: Patterns of regional hydrological variability in central-southern Altiplano (18–26∘ S) lakes during the last 500 years, Palaeogeogr. Palaeocl., 194, 319–338, https://doi.org/10.1016/S0031-0182(03)00284-0, 2003.
Vignoni, P. A., Jurikova, H, Plessen, B., Tjallingii, R., Córdoba, F. E., Liebetrau, V., Lecomte, K. L., Pinkerneil, S., Grudzinska, I., Schleicher, A., Viotto, S., Santamans, C., Rae, J. W. B., and Brauer, A.: Geochemistry of a high-altitude hypersaline Andean lake and associated carbonate deposits (Laguna del Peinado, Southern Puna Plateau, NW Argentina), IAL IPA Joint Meeting 2022, San Carlos de Bariloche, Argentina, 27 November–1 December 2022, FS16-135, https://doi.org/10.5281/zenodo.7305148, 2022.
Villafañe, P. G., Cónsole-Gonella, C., Cury, L. F., and Farías, M. E.: Short-term microbialite resurgence as indicator of ecological resilience against crises (Catamarca, Argentine Puna), Environ. Microbiol. Rep., 13, 659–667, https://doi.org/10.1111/1758-2229.12977, 2021.
Vuille, M. and Ammann, C.: Regional snowfall patterns in the high, arid Andes, Climatic Change, 36, 413–423, https://doi.org/10.1029/2000GL011871, 1997.
Vuille, M., Burns, S. J., Taylor, B. L., Cruz, F. W., Bird, B. W., Abbott, M. B., Kanner, L. C., Cheng, H., and Novello, V. F.: A review of the South American monsoon history as recorded in stable isotopic proxies over the past two millennia, Clim. Past, 8, 1309–1321, https://doi.org/10.5194/cp-8-1309-2012, 2012.
Ward, K. M., Delph, J. R., Zandt, G., Beck, S. L., and Ducea, M. N.: Magmatic evolution of a Cordilleran flare-up and its role in the creation of silicic crust, Sci. Rep., 7, 1–8, https://doi.org/10.1038/s41598-017-09015-5, 2017.
Yu, S.-Y., Shen, J., and Colman, S. M.: Modeling the Radiocarbon Reservoir Effect in Lacustrine Systems, Radiocarbon, 49, 1241–1254, https://doi.org/10.1017/S0033822200043150, 2007.
Zech, R., May, J.-H., Kull, C., Ilgner, J., Kubik, P. W., and Veit, H.: Timing of the late Quaternary glaciation in the Andes from ∼15 to 40∘ S, J. Quaternary Sci., 23, 635–647, https://doi.org/10.1002/jqs.1200, 2008.
Zhou, K., Xu, H., Lan, J., Yan, D., Sheng, E., Yu, K., Song, Y., Zhang, J., Fu, P., and Xu, S.: Variable Late Holocene 14C Reservoir Ages in Lake Bosten, Northwestern China, Front. Earth Sci., 7, 1–11, https://doi.org/10.3389/feart.2019.00328, 2020.