the Creative Commons Attribution 4.0 License.
the Creative Commons Attribution 4.0 License.
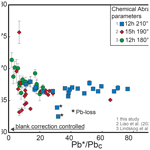
Short communication: Resolving the discrepancy between U–Pb age estimates for the “Likhall” bed, a key level in the Ordovician timescale
Anders Lindskog
Urs Schaltegger
The “Likhall” bed is a rare case of a single-age zircon population from a carbonate rock, which in this case is contextualised with remarkable biotic and environmental changes and with meteorite bombardment of Earth after an asteroid breakup in space. Published high-precision chemical abrasion isotope dilution thermal ionisation mass spectrometry (CA-ID-TIMS) U–Pb age estimates disagree on the typical precision of <0.1 % for a date, which has led to discrepancies in the interpretation of the timing of events and their possible cause–effect relationships. Here we evaluate the relative strengths, weaknesses, and discrepancies in the datasets published so far; propose strategies to overcome them; and present a new U–Pb dataset with improved precision and accuracy. Ultimately, we find that domains of residual Pb loss are a significant source of age offset between previously published data, amplified by differences in data evaluation strategies. Our new dataset benefits from an improved chemical abrasion protocol resulting in a more complete mitigation of decay-damage-induced grain portions and points to a weighted mean age estimate of 466.37±0.14/0.18/0.53 Ma for the “Likhall” zircon population. This age is intermediate between previous estimates but outside of analytical uncertainty, and it provides a firm tie point for the Ordovician timescale.
- Article
(1437 KB) - Full-text XML
-
Supplement
(327 KB) - BibTeX
- EndNote
The Ordovician “Likhall” bed hosts zircons and fossils and is an “orthoceratite limestone” outcropping at Kinnekulle, Sweden, locally referred to as “Täljsten”, which is an important marker interval in the regional stratigraphy (see below). These strata record remarkable changes in palaeoenvironmental conditions and biodiversity (Lindskog and Eriksson, 2017; Servais and Harper, 2018; Lindskog et al., 2017). Besides being unusually rich in prismatic zircon of apparently single age, the “Likhall” bed coincides with an interval with uniquely abundant L chondritic “fossil” meteorites and purportedly related chromite grains (Lindskog et al., 2017; Liao et al., 2020; and references therein). This has been linked to the breakup of an asteroid in space, and temporal overlap between excess meteoritic matter and prominent climatic changes and biodiversity peaks has spurred the controversial hypothesis that meteorite bombardment fundamentally affected the Ordovician climate and instigated biodiversification (Schmitz et al., 2008, 2019; Lindskog et al., 2017; Rasmussen et al., 2021). Considering absolute and/or numerical time perspectives, two individual U–Pb geochronological studies have arrived at significantly different age estimates of zircon from the “Likhall” bed – 467.50±0.28 (Lindskog et al., 2017) and 465.18±0.17 Ma (Liao et al., 2020) – each reaching contrasting conclusions for the absolute timing of the L chondrite breakup event and with differing implications for the Ordovician event stratigraphy and timescales (particularly the Darriwilian Stage).
While the occurrence of an apparently single U–Pb zircon age population in a carbonate rock is already uncommon, the percent difference between these two previously published 206Pb–238U ages amounts to 0.4 %, which exceeds the expected reproducibility level of, for example, natural zircon reference materials (0.1 %; Schaltegger et al., 2021). Thus, we have to evaluate the accuracy of the published U–Pb ages on the “Likhall” zircons before we can determine a more robust age estimate. A significant effort has recently been undertaken by the U–Pb community to reduce inter-lab bias in isotope dilution thermal ionisation mass spectrometry (ID-TIMS) via the use of precisely calibrated EARTHTIME (ET) isotopic tracers (Condon et al., 2015; McLean et al., 2015), community-wide shared data reduction (Bowring et al., 2012; McLean et al., 2011), and sample preparation procedures (Widmann et al., 2019). However, the two previously published “Likhall” zircon datasets were produced employing different isotopic tracers, instrumentation, and chemical abrasion procedures: a mixed ET 202Pb–205Pb–233U–235U tracer was used in the Lindskog et al. (2017) study, while an in-house 202Pb–205Pb–233U–236U tracer was used for the Liao et al. (2020) study. Further differences concern the measurement procedure of the U isotope composition (using TIMS as UO2 for the first and using multi-collector inductively coupled plasma mass spectrometry (MC-ICP-MS) as a metal for the latter). Most importantly, the chemical abrasion procedures also differ, with annealing at 900 °C for 3 d and leaching at 180 °C for 12 h (Lindskog et al., 2017) and annealing at 900 °C for 48 h and leaching at 190 °C for 15 h (Liao et al., 2020).
The variation in the chemical abrasion procedure parameters has profound effects on the U–Pb zircon isotopic systematics of the analysed material. The duration and the temperature of the partial dissolution may have a significant impact on the potential to effectively remove structurally damaged domains, which typically produce anomalously young dates (Mattinson, 2005; Huyskens et al., 2016; Keller et al., 2019; Widmann et al., 2019). Both of the aforementioned studies of the “Likhall” bed utilised temperatures lower than the most recent recommendation for the chemical abrasion procedure (McKanna et al., 2023, 2024; Widmann et al., 2019), which raises concerns about remnant Pb loss domains present in the zircons analysed. Different chemical abrasion procedures may be variably effective in removing visible inclusions (e.g. apatite and/or melt inclusions) in the analysed zircon crystals.
The impact of tracer uncertainty is significantly lower than the 0.4 % discrepancy between the absolute ages obtained from “Likhall” bed. Similarly, the variation in the instrumental setup for U isotope analysis does not seem to introduce significant offsets, although we cannot evaluate this with certainty due to the limited amount of comparable data. The age of the natural reference zircon material TEMORA reported by Liao et al. (2020) from the mixed TIMS–MC-ICP-MS analysis (417.19±0.15 Ma) is in agreement with the most recent estimates of 417.310±0.074 Ma (von Quadt et al., 2016) and 417.353±0.052 Ma (Schaltegger et al., 2021). Reported values of the ET100 solution from the lab utilised in Liao et al. (2020) is 100.169±0.047 Ma, indistinguishable from the reference value of 100.173±0.003 Ma (Schaltegger et al., 2021) and our value (100.1678±0.0046 Ma) produced during the period of data collection of this study.
Thus, we can reasonably assume that the difference in the chemical abrasion procedure is the main source of the significant difference between the two previously published studies of “Likhall” zircons. Furthermore, there is a difference in the data interpretation strategy of the two published datasets. Lindskog et al. (2017) put the emphasis on the largest statistically valid weighted mean age plateau, whereas Liao et al. (2020) chose the youngest cluster of zircon U–Pb analyses. In the following, we will first explore different interpretation strategies of the existing data, such as looking for (i) the largest statistically valid plateau, (ii) the youngest cluster, (iii) the duration of time recorded by the youngest and oldest zircon U–Pb date (Δt), and (iv) the youngest concordant single zircon U–Pb analysis. Ideally, applying the same interpretation methodology to both datasets should reduce the discrepancy of the absolute U–Pb ages. Furthermore, we add another set of U–Pb dates from the same material but apply the chemical abrasion procedure of Widmann et al. (2019), which should more effectively mitigate Pb loss. Ultimately, we will suggest a revised age for the “Likhall” bed.
Single grains of “Likhall” zircon crystals free of visible inclusions and cracks were hand-picked using a binocular microscope at a magnification of ×20 to ×40 from the same Petri dish as the material analysed by Lindskog et al. (2017). The size of individual zircons was variable, with lengths ranging from ∼50 to ∼300 µm. The chemical abrasion procedures were 48 h of annealing at 900 °C and 12 h partial dissolution at 210 °C, as defined as optimal in Widmann et al. (2019). Afterwards, we observed that some zircons had either fragmented, or embayment was predominantly at the core, or some channels had more efficiently progressed inwards, without any systematic preference identifiable. Individual zircon grains were washed in 3 mL Savillex beakers in an ultrasonic bath, washed 4 times in 7N HNO3, transferred into individual 200 µL Savillex microcapsules along with 2–3 drops of HFconc and 3.9–5.5 mg of a mixed 202Pb–205Pb–233U–235U tracer solution (ET2535; Condon et al., 2015; McLean et al., 2015), and dissolved at 210 °C in a Parr bomb for 48 h. After dissolution, samples were dried down on a hotplate at 120 °C and re-dissolved in 3N HCl, and then U and Pb were separated using a single-column anion exchange chemistry. Uranium and Pb were loaded on outgassed, zone-refined, single Re filaments with a silica gel/phosphoric acid emitter solution (Gerstenberger and Haase, 1997). Uranium and Pb isotopic compositions were measured on an Isotopx Phoenix TIMS instrument at the University of Geneva. Lead was measured in dynamic mode using a Daly detector, and U was measured as an oxide in static mode using Faraday cups coupled to 1012Ω resistance amplifiers. Measured isotopic ratios were corrected for interferences of 238U18O16O on 235U16O2 using an composition of 0.00205, based on repeat measurements of the U500 standard. Mass fractionation of U was corrected using a double-isotope tracer with a of 0.99506±0.005. Mass fractionation of Pb was calculated and corrected using a ratio of 0.99924±0.00027 (1σ) (Condon et al., 2015). Zircon Pb analyses were corrected for laboratory blanks, with a of 17.10±0.21, a of 15.07±0.11, and a of 36.17±0.25 (all 1σ), based on repeat measurements of total procedural blanks for the zircon U–Pb column chemistry. All data were processed using the Tripoli v. 4.10 and Redux v. 3.7.1 U–Pb software (Bowring et al., 2012; McLean et al., 2011). Weighted mean U–Pb age uncertainties are reported at the 2σ level in the format , where A is the weighted mean age; X is analytical uncertainty; Y is analytical and tracer uncertainty combined; and Z is analytical, tracer, and decay constant uncertainties combined (Schoene et al., 2006). Thorium abundances for each grain, and subsequently , were calculated using the abundance of 208Pb within the crystal and the age to calculate radiogenic in-growth (McLean et al., 2011). All U–Pb dates were corrected for initial 230Th disequilibrium, assuming a ratio of the magma of 3.5±1.
Repeat analyses of the ET100 solution ( date: 100.173±0.003 Ma; Schaltegger et al., 2021) yielded a value of 100.1678±0.0046 Ma (MSWD = 3.2, ) during the period of data collection. The eight rejected ET100 samples were prepared and analysed consecutively, yielding an anomalously young average age, a detail observed by previous studies (Schaltegger et al., 2021).
The Baltoscandian region was largely covered by an epeiric sea throughout much of the Ordovician, leaving behind a sedimentary record mainly in the form of mudstones, shales, and limestones (e.g. Nielsen et al., 2023, and references therein). In the Lower–Middle Ordovician, the continent Baltica was at a relatively high latitudinal position, and any relief of the regional Precambrian basement was nearly completely smoothed out. These conditions limited terrigenous input into the basin, and sedimentation rates were typically low (a few mm ka−1). The regional intra-cratonic Ordovician succession is therefore relatively thin. Volcanic dust may have contributed a relatively large proportion of the non-carbonate sedimentary materials (Lindstrom, 1974). The Middle Ordovician (Dapingian–Darriwilian) was mainly characterised by widespread deposition of cool-water carbonate sediments, commonly referred to as “orthoceratite limestone” in Sweden (e.g. Lindskog and Eriksson, 2017; Lindskog et al., 2023).
The Ordovician succession of Baltoscandia contains numerous bentonite beds of varying thickness and lateral distribution, the most prominent of which occur in the Upper Ordovician (e.g. Ballo et al., 2019; Bergström, 1989; and references therein). Few of the bentonite beds have been isotopically dated and even fewer using modern techniques such as chemical abrasion isotope dilution thermal ionisation mass spectrometry (CA-ID-TIMS). Some carbonate beds in the “orthoceratite limestone” contain abundant zircon, and the crystal characteristics and U–Pb age data of the grains indicate a volcanic origin (Lindskog et al., 2017; Liao et al., 2020). Thus, the zircon-rich beds arguably represent “crypto-tephra” (McLaughlin et al., 2023).
The table mountain Kinnekulle in the province of Västergötland, central southern Sweden, hosts a relatively expanded “orthoceratite limestone” succession (e.g. Lindskog and Eriksson, 2017). These rocks have been the target of several studies of palaeontology, sedimentology, and geochemistry (e.g. Ahlberg et al., 2023, and references therein). Our sample materials are derived from the Thorsberg quarry on eastern Kinnekulle (WGS84 coordinates 58.579167, 13.429444), from a distinct grey interval traditionally referred to as the “Täljsten” (“carving stone”) by local quarry workers. In more detail, the sample is derived from a specific bed referred to as “Likhall” (“corpse slab”), which has been shown to contain very abundant zircon grains (Lindskog et al., 2017; Liao et al., 2020). The biostratigraphic context of this ca. 10 cm thick bed is very well known, and its base coincides with that of the geographically widely distributed Yangtzeplacognathus crassus conodont zone in the middle Darriwilian (for more details, see Lindskog et al., 2017).
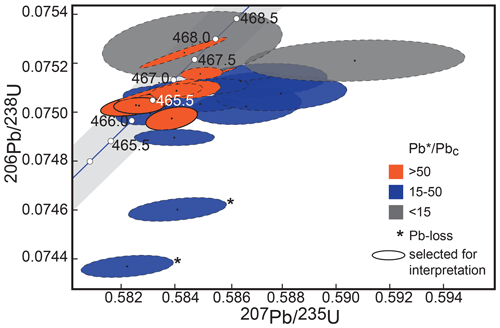
Figure 1Concordia diagram generated in ETRedux v. 3.7.1 using colour-coding for bands of , as discussed in the text, for the error ellipses. Orange error ellipses indicate >50, blue error ellipses indicate values between 15–50, and grey error ellipses indicate <15. Ellipses marked by an asterisk are interpreted to be affected by Pb loss. Black outlines indicate analyses considered for the weighted mean age, where the selection criteria are >50 and analytical concordance (partial or full ellipsis overlaps with the uncertainty band of the concordia curve). The grey band illustrates the uncertainty band of the concordia curve.
Table 1U–Pb ID-TIMS measurement results of individual zircons from the “Likhall” bed.
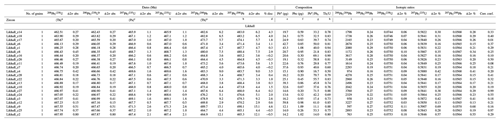
a Corrected for initial disequilibrium using radiogenic 208Pb and [magma] = 3.5±1.
b Isotopic dates calculated using (Jaffey et al., 1971) and (Jaffey et al., 1971).
c Corrected for initial disequilibrium using initial fraction activity ratio .
d % discordance = .
e Total mass of radiogenic Pb.
f Total mass of common Pb.
g Ratio of radiogenic Pb (including 208Pb) to common Pb.
h Th contents calculated from radiogenic 208Pb and 230Th-corrected date of the sample, assuming concordance between U–Pb and Th–Pb systems.
i Measured ratio corrected for fractionation and spike contribution only.
j Measured ratios corrected for fractionation, tracer, and blank.
A total of 22 zircons from the zircon-rich “Likhall” bed were analysed for their U–Pb isotopic composition. The U and Pb isotopic data are presented in Table 1, and interpreted U–Pb dates are illustrated in concordia space in Fig. 1, revealing a large spread in dates and variable discordance. The new data presented here comprise 15 analytically concordant and 7 discordant zircon analyses, which range in dates from 468.8±1.2 to 462.43±0.27 Ma. The ratio of radiogenic to common lead () ranges from 11.1 to 79.7, with variable amounts of Pbc (0.20 to 1.09 pg). The dates range from 461±10 to 490±15 Ma.
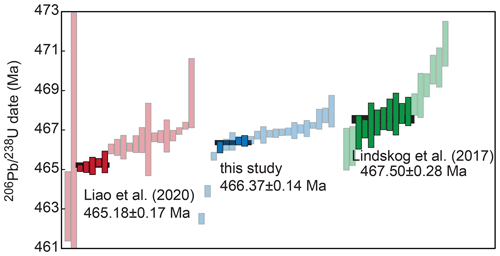
Figure 2Rank-order plot of calculated /single zircon U–Pb dates from Liao et al. (2020), this study, and Lindskog et al. (2017). Transparent bars were not considered for the calculation of the weighted mean age, as per each study's selection criteria (see text for more details).
The compilation of previously published zircon U–Pb analyses of the “Likhall” bed comprises 17 analyses from Lindskog et al. (2017) and 21 analyses from Liao et al. (2020), which are now complemented by our 22 new analyses in this study. The dates of all these datasets are plotted in Fig. 2, along with the weighted mean age for each of the datasets following the selection criteria discussed below. All data are available in Table 1 and in the Supplement (Table S1).
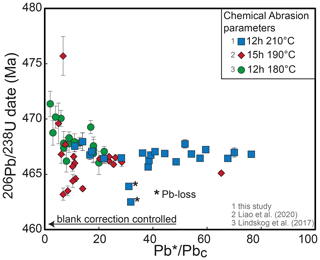
Figure 3Comparison of calculated zircon dates with their respective values. Low values typically indicate stronger effects of blank correction on the calculated dates (Schaltegger et al., 2021). Colours indicate the different studies and differences in their chemical abrasion procedures. Samples marked with an asterisk are discordant in concordia space (see Fig. 1).
5.1 Radiogenic Pb common Pb ratio () as a selection criterion
The colour-coding of the data ellipses in Fig. 1 indicates that the majority of the analyses plot onto or near concordia within its uncertainty band. Analyses with low (< 50) have significantly higher scatter in both and ratios. Subsequently, we only use high- (>50) analyses for our age interpretation and will also apply this strategy to the previously published datasets. The accuracy of dates is higher at >15 (Schaltegger et al., 2021), which is the case for most of our analyses that underwent chemical abrasion for 12 h at 210 °C and for some of the analyses of Liao et al. (2020) abraded for 15 h at 190 °C (Fig. 3). Contrastingly, a major part of the Lindskog et al. (2017) dates abraded for 12 h at 180 °C plots below this threshold at variable dates. Considering the significant differences in the range of reported in the three datasets, we have also evaluated bands ranging <15 and 15–50 in addition to >50 discussed above.
When considering only analyses with , the datasets of Lindskog et al. (2017), Liao et al. (2020), and this study would result in weighted mean ages of 468.40±0.50 Ma (MSWD = 2.7, n=10), 465.92±0.21 Ma (MSWD = 3.6, n=14), and 467.67±0.84 Ma (MSWD = 0.18, n=2). Improvement in the weighted mean ages between the studies can be observed when considering band 15–50, where the studies result in 467.12±0.59 Ma (MSWD = 0.46, n=6), 466.68±0.18 Ma (MSWD = 5.4, n=6), and 466.79±0.15 Ma (MSWD = 1.4, n=11) (discordant data not considered; Table S1). In our new dataset, the discrepancy between the proposed U–Pb age when comparing bands 15–50 and >50 is 0.09 %, which is the expected reproducibility of natural reference materials (Schaltegger et al., 2021). Potential sources of this discrepancy may be (i) residual Pb loss, (ii) inaccurate Pb-blank correction, or (iii) natural variability and duration of magmatic zircon growth in a magma chamber. Where possible, high- data should be preferred for interpretation to reduce effects from potential (i) residual Pb loss and (ii) inaccurate Pb-blank correction.
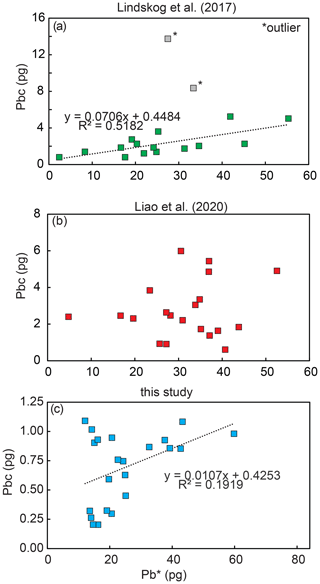
Figure 4Comparison of Pbc vs. Pb∗ of (a) the Lindskog et al. (2017) study, (b) the Liao et al. (2020) study, and (c) this study. No study exhibits simple correlation without outlier rejection. A positive correlation between Pbc and Pb∗ may indicate the presence of inclusions (mineral and/or melt), which were not removed during the chemical abrasion process.
Adopting the (>50 in this study) as a criterion for data selection is thus an important step towards greater accuracy. The data of Lindskog et al. (2017) show significant correlation between Pb∗ and Pbc (Fig. 4) at overall high Pbc values (up to ∼6 pg). This may indicate that some indiscernible mineral inclusions were not removed during chemical abrasion. Chemical abrasion at higher temperatures should access and dissolve these (McKanna et al., 2023), if the hypothesis of unresolved inclusions is correct, and would reduce the total Pbc observed. Subsequently, we would then use only high- (>50) analyses to make an age interpretation.
The high Pbc concentrations of the Lindskog et al. (2017) analyses (up to 6 pg) suggest that the conditions during the chemical abrasion procedure were not sufficient to remove the inclusions that are clearly visible in the grain separate (Lindskog et al., 2017), leading to generally low . The higher dependency on the Pbc correction can potentially result in over- or under-correction, which results in younger or older dates calculated. This is further explored in Sect. 5.3.
5.2 Residual Pb loss in chemically abraded natural zircon
The effect of Pb loss may be mitigated by removing decay-damaged (metamict) portions of the zircon grains that have undergone open-system behaviour, using the chemical abrasion procedure (Mattinson, 2005; Mezger and Krogstad, 1997). Subsequently, if all Pb loss domains were removed, the isotopic analysis should yield a concordant result for both the 206Pb–238U and 207Pb–235U decay series. However, the chemical abrasion may not have removed 100 % of the metamict portions, and some domains with partial Pb loss may still be present in the grain (so-called residual Pb loss). This may be the case even if the most recent calibration of the chemical abrasion procedure (12 h at 210 °C; Widmann et al., 2019) is utilised. It should also be noted that chemical abrasion calibration may be affected by geochemical composition, parent isotope zonation, accessibility of damaged domains for the chemical agent, and other effects. Previous work has shown that natural zircon reference materials (TEMORA and GJ-1) treated at 180 and 210 °C for 12 h retain excess scatter in their U–Pb systematics, restricting repeatability confidence to ca. 0.1 % of the absolute age, while synthetic solutions offer a repeatability at a precision of up to 0.01 % in the same study (Schaltegger et al., 2021). It is, however, not clear if excess scatter in TEMORA and GJ-1 is derived from inherited older components, magmatic processes, or residual Pb loss for these materials (Schaltegger et al., 2021). When comparing the effects of different chemical abrasion temperatures, Huyskens et al. (2016) found that temperatures of 190 °C and lower may yield incomplete removal of Pb loss domains, in agreement with later experiments (e.g. Widmann et al., 2019; Schaltegger et al., 2021). Such an effect of incomplete removal of metamict domains biased by Pb loss may possibly be detected through analytical discordance between the two decay schemes (Condon et al., 2024), provided that the analytical precision, especially of the decay series, was sufficient, assuming that no inheritance is present (possible in the new dataset, not resolvable in the dataset of Lindskog et al., 2017).
Between the previously published age estimates for the “Likhall” bed by Lindskog et al. (2017) (467.50±0.28 Ma) and Liao et al. (2020) (465.18±0.17 Ma), there is a discrepancy of ca. 0.4 % between the proposed U–Pb ages (Fig. 2). The two studies differ in their chemical abrasion protocols: Lindskog et al. (2017) utilised a 180 °C and 12 h procedure, whereas Liao et al. (2020) utilised a 190 °C and 15 h procedure. Both of these protocols diverge from the Widmann et al. (2019) parameters and we must consider the potential that these analyses included relict domains of Pb loss, that may bias the U–Pb dates towards younger dates for both studies. Curiously, for the first case, the lower T abrasion resulted in (on average) older interpreted zircon U–Pb data (Lindskog et al., 2017), an effect we will explore in the next section discussing blank corrections.
Both the Lindskog et al. (2017) and Liao et al. (2020) datasets show very little evidence for normal discordance, as analyses which are discordant are typically older than the interpreted U–Pb age, suggesting inheritance rather than Pb loss. Negative discordance can be masked if the analytical precision is insufficient, in particular when the measured 207Pb intensity is low. In our new data we observe two discordant analyses that are by ∼2–4 Ma (Fig. 1) younger than the previous age estimates (Lindskog et al., 2017; Liao et al., 2020). We therefore infer that the data of Liao et al. (2020) and Lindskog et al. (2017) were affected by Pb loss domains and/or relict inclusions that were not penetrated during chemical abrasion (expressed through elevated Pbc and/or younger dates), which may also not be excluded for our new dataset with absolute certainty. Relict inclusions have an unknown amount of Pbc, and its composition cannot be assessed by ID-TIMS data. This could result in erroneous blank composition corrections, which may result in older U–Pb dates. Therefore, we base our discussion and interpretation on those data that are the least affected by Pb-blank correction.
5.3 Lead-blank isotopic composition correction effects on the spread of zircon U–Pb dates
One of the fundamental assumptions in zircon U–Pb geochronology is that zircon does not incorporate Pb during crystallisation and therefore does not contain initial Pb (which can be monitored through analysis of 204Pb) (Watson et al., 1997). Therefore, all zircon U–Pb analyses can be corrected for the presence of common Pb through measurement of 204Pb during data acquisition, assuming that all Pbc is derived from the procedural blank, by adopting the mean isotopic composition from repeat analysis of procedural blank measurements. The uncertainty of this mean blank isotopic composition is propagated into the U–Pb date calculation (Schmitz and Schoene, 2007). An accurate and precise correction of blank Pb thus results in more precise and accurate U–Pb dates. Conversely, inaccurate blank corrections may result in U–Pb dates that are apparently too old or too young if the is low (Schaltegger et al., 2021).
In the Lindskog et al. (2017) dataset, a significant proportion of the analytical uncertainty is controlled by the Pb-blank composition correction, as ratios range from 2 to 22, while the Liao et al. (2020) dataset ranges from 2 to 30 (plus one high value at 67). In our new dataset, we observe from 11 to 78. When we compare the zircon U–Pb dates with their (Fig. 3), we observe that the Lindskog et al. (2017) data exhibit a slightly negative correlation, whereas the Liao et al. (2020) data are scattered. However, we observe in the Liao et al. (2020) data that the youngest analyses are consistently associated with lower , with the exception of the analysis with the highest , which is also relatively young (Fig. 3). In our newly acquired dataset, correlation between the zircon U–Pb date and its is absent, suggesting that blank correction does not introduce significant bias (Fig. 3).
The strong correlation between Pb∗ and Pbc (R2 of 0.52 after rejecting two outliers; Pb∗ max = 55.33 pg and Pbc max = 5.24 pg) in the Lindskog et al. (2017) dataset is concerning (Fig. 4), as it implies that the analysed zircons contained inclusions that were not removed during the chemical abrasion procedure. Evidence for potential inclusions (before chemical abrasion) is provided by imaging of zircon crystals analysed by Lindskog et al. (2017) and Liao et al. (2020), matching our observations during mineral selection. If we assume that larger zircons contain more Pb∗ and a larger volume of Pbc-bearing inclusions, this would explain why the Lindskog et al. (2017) dataset exhibits a negative correlation between and dates, as they are more strongly affected by an inaccurate blank correction. The slope of the correlation between and dates is controlled by the difference between the “true” Pbc composition and the assigned Pbc composition for blank correction. In the Liao et al. (2020) dataset, correlation between Pb∗ and Pbc is absent, but measured are comparable to those of Lindskog et al. (2017; Fig. 4). Our new dataset does not show any correlation between Pb∗ and Pbc (Pb∗ max = 59.80 pg and Pbc max = 1.09 pg; Fig. 4) or between and date (Fig. 3).
Some of the low-Pb∗ zircons in our new data also have elevated blanks, causing scatter in the low-Pb∗ analysis systematics, whereas all the high-Pb∗ analyses associate with slightly higher blanks. We therefore consider it likely that the Pbc is primarily controlled by unresolved inclusion (transparent, mineral, and/or melt). These observations are in line with the observations by McKanna et al. (2023) and with the Lindskog et al. (2017) data (Fig. 4a), that chemical abrasion at high temperatures is necessary to effectively remove inclusions that are deeply seated within the zircon crystal. Factors such as location of inclusions within or outside of damaged high-U domains may impact the efficiency of the chemical abrasion procedure to remove inclusions.
5.4 The impact of the interpretation strategy on U–Pb zircon ages
Correlation between U–Pb dates and has implications for interpreting the absolute age of the “Likhall” bed. Several different interpretation strategies exist, such as (i) the weighted mean of a subset of data (e.g. Lindskog et al., 2017), (ii) the youngest cluster of overlapping analyses at 2σ (e.g. Liao et al., 2020), (iii) regarding the entire range of concordant zircon U–Pb analyses as autocrystic growth within the magma chamber (Samperton et al., 2015), (iv) regarding the youngest concordant analysis as best proxy for the timing of eruption, and (v) applying a stochastic (Bayesian) sampling approach (Keller et al., 2018; Traylor et al., 2024). We discuss in the following the impact of interpretation scenarios i–iv on the suggested age for the “Likhall” bed (v is beyond the scope of this study, but we report model results in Table S2, which we find to be too heavily dependent on the input data). Analytical and mineralogical effects such as using variable or inaccurate blank isotopic composition and the presence of Pbc-rich inclusions, together with the presence of residual Pb loss and minor inheritance of old radiogenic Pb (potentially from inherited cores), add to any of the further discussed discrepancies.
5.4.1 (i) Subset interpretation
Lindskog et al. (2017) preferred a data interpretation based on the statistically most robust weighted mean age, representing the largest number of statistically valid analyses, which results in 467.50±0.28 Ma (MSWD = 1.4, n=9; published value rejecting two younger and six older, concordant analyses). The underlying assumption is that some unresolved Pb loss may affect the youngest analyses and that inheritance may explain the oldest range of zircons analysed (Samperton et al., 2015). Applying the same strategy to the Liao et al. (2020) dataset, we obtain 466.34±0.20 Ma (MSWD = 0.99, n=12), rejecting five younger and three older analyses. For our new dataset, the result would be 466.875±0.074 Ma (MSWD = 0.87, n=9), rejecting three discordant analyses, six younger analyses, and five older analyses. The maximum difference between the absolute ages amounts to 0.25 %, which is better than the difference of 0.4 % in the previously published values. The lowest discrepancy is achieved between Liao et al. (2020) and our dataset, with a difference of 0.11 %.
5.4.2 (ii) Youngest cluster interpretation
Liao et al. (2020) preferred an interpretation based on the youngest statistically valid age cluster, which resulted in 465.18±0.17 Ma (MSWD = 1.3, n=5; published value). The underlying assumption is that all Pb loss is effectively removed and that inheritance or protracted growth in the magma chamber is responsible for the older analyses. For the Lindskog et al. (2017) data, this approach results in 466.96±0.30 Ma (MSWD = 1.5, n=6). For our new dataset, the corresponding result is 466.43±0.11 Ma (MSWD = 5, n=5). The resulting maximum spread is 0.38 %, close to the published discrepancy of 0.4 %. The lowest discrepancy is achieved between Lindskog et al. (2017) and our dataset, with a difference of 0.11 %.
5.4.3 (iii) Range of concordant zircon U–Pb dates
The duration of zircon crystallisation in a magma chamber is of interest for studies involving magma chamber dynamics and crustal evolution, and here we compare the Δt defined as spread between the youngest and oldest (concordant) zircon U–Pb analysis. The underlying assumption is that all concordant analyses reflect growth from the same magma chamber (Samperton et al., 2015). The Δt is 5.35 Ma for the Lindskog dataset, 3.8 Ma for Liao et al. (2020) (rejecting two outliers marked by Liao et al., 2020), and 1.82 Ma for our new dataset (rejecting three young discordant data points) – results which differ significantly from each other. One possible explanation could be the unresolved inclusions and resultant low , which can result in calculated dates that are too young or too old, augmenting natural spreads in zircon U–Pb dates. The maximum difference between the three datasets is 194 %, suggesting that interpretation is problematic with respect to the duration of magma chamber activity.
5.4.4 (iv) Youngest concordant zircon U–Pb analysis
The youngest concordant zircon U–Pb analysis interpretation can be useful in volcanic samples. Here, the base assumption is that zircon continuously crystallises in the magma, and the youngest zircon represents the best proxy to the timing of eruption. In this regard, for Liao et al. (2020) the youngest concordant zircon U–Pb analysis is 465.07±0.17 Ma, for Lindskog et al. (2017) it is 466.03±1.06 Ma, and for our new dataset it is 466.05±0.29 Ma. The maximum difference between the three datasets is 0.21 %, better than the discrepancy of the published U–Pb dates. There is a near-indistinguishable difference between the Lindskog et al. (2017) data and our youngest zircon U–Pb analyses (0.004 %).
5.5 Which strategy to choose?
It becomes clear from Fig. 2 that the three datasets were obtained under different analytical regimes in different labs. Among the differences is an improvement in analytical precision (increase in through blank reduction). This will help to narrow down the duration of zircon growth in the magma system prior to eruption and leads to more reliable identification of autocrystic vs. antecrystic zircon. Identifying Pb loss remains challenging in the older datasets, which utilised partial dissolution procedures at lower temperatures and/or of shorter duration. Furthermore, low ratios of obscure discordance between the two decay series due to elevated uncertainty. The largest difference in age, however, is caused by the varying approaches to data interpretation. If the same strategy is chosen, the discrepancy between the proposed U–Pb age in the two previously published studies and the present one significantly decreases.
We can conclude that is of fundamental importance for the “Likhall” zircon datasets. If we, for example, consider only analyses that are characterised by >50 – i.e. a value where zircon dates are barely affected by blank correction – we would have to reject the entire Lindskog et al. (2017) dataset and all but 1 analysis from the Liao et al. (2020) study, and this leaves only 8 out of 22 analyses from our new dataset, of which only 3 form a cluster of youngest overlapping grains. Adopting this reduced dataset for interpretation yields the following results: (i) the largest number of overlapping U–Pb dates forms a weighted mean of 466.76±0.12 Ma; (ii) the youngest cluster is 466.37±0.14 Ma; (iii) the Δt reduces to 780 kyr, which is more consistent with predictions of thermal models for magma chambers (e.g. Caricchi et al., 2016; Weber et al., 2020); and (iv) the youngest concordant zircon analysis remains unchanged at 466.05±0.29 Ma. The maximum difference between interpretations (i), (ii), and (iv) is 0.15 %, close to the desired reproducibility value of 0.1 % for natural reference materials (Schaltegger et al., 2021). More rigorous filtering based on thus yields a more coherent dataset between the three studies, however, at low n value.
Therefore, we propose that the weighted mean age of the youngest cluster of three high- analyses at 466.37±0.14/0.18/0.53 Ma (analytical/+ tracer/+ decay constant uncertainty; MSWD = 1.9, n=3) has the highest probability of being an accurate age estimate for the “Likhall” bed.
5.6 Implications for the Ordovician timescale and the absolute timing of events
Our new age for the “Likhall” bed provides a carefully considered and well-constrained tie point in the overall Ordovician timescale and event-stratigraphic framework. In Geologic Time Scale 2020 (GTS2020), the level corresponding to “Likhall”, i.e. the basal Yangtzeplacognathus crassus conodont zone, is placed at ca. 469 Ma (Goldman et al., 2020; their Fig. 20.3). This is well outside our new age estimate of 466.37±0.14/0.18/0.53 Ma (and also those of Lindskog et al., 2017, and Liao et al., 2020) and thus warrants adjustment of the Ordovician timescale – especially given that the range of the Y. crassus zone in the GTS2020 scheme does not even overlap with our age estimate. The revised age for “Likhall” further suggests that the timing of the L chondrite breakup event in space, as interpreted based on cosmic-ray exposure age data from “fossil” meteorites, should be placed at ca. 467 Ma (Korochantseva et al., 2007; Heck et al., 2008; Lindskog et al., 2017; Liao et al., 2020; and references therein).
We refrain from extending our data interpretations until reliable U–Pb dates from both older and younger strata are available. Nonetheless, it is apparent from the relative timing in the rock record that the purported meteorite bombardment significantly postdates the onset of climate change and biodiversity spurts in the Middle Ordovician (e.g. Lindskog et al., 2017, 2023; Rasmussen et al., 2021). These stratigraphic offsets are unlikely to be bridged by numerical dating, but continued efforts to refine the Ordovician timescale will provide essential temporal context to the long-term development of the geobiosphere.
-
Our new high-precision dataset of the “Likhall” zircon population represents an analytical improvement over previously published results: (a) our new data show higher and are therefore less affected by the choice of isotopic composition used for blank correction, (b) higher analytical precision allows the identification of normal discordance in young analyses, and (c) the presence of residual Pb loss despite application of the currently considered optimal parameters for chemical abrasion (210 °C for 12 h; Widmann et al., 2019) suggests that previous data acquired at lower chemical abrasion temperatures were (likely more) affected by Pb loss domains. The elevated Pbc in the former studies is suggested to be due to the incomplete removal of Pbc-rich inclusions during chemical abrasion.
-
The choice of the data interpretation strategy is the main reason for the discrepancies between datasets from the three different laboratories (Lindskog et al., 2017; Liao et al., 2020; and the present study), particularly in cases where elevated Pbc causes decreased analytical precision. Further reduction in the reproducibility between these studies is caused by the use of different tracer solutions and their respective calibrations.
-
The difference in interpreted age drastically decreases when only data subsets with high are considered. For the datasets considered here, this implies an empirical threshold of 50. For analyses with >50, the weighted mean (youngest cluster) U–Pb zircon age for the “Likhall” bed is 466.37±0.14/0.18/0.53 (analytical/+ tracer/+ decay constant uncertainties), suggesting that previously published U–Pb ages are inaccurate.
-
The efficient removal of Pb loss domains and micro-inclusions is of paramount importance for achieving an accurate U–Pb date. Considering the presence of discordant, young analyses in our Ordovician-age dataset despite chemical abrasion conditions that are regarded as being optimal, there is incentive to further develop the chemical abrasion procedure.
-
Considering biostratigraphic aspects, compared to GTS2020, the revised age of the “Likhall” bed necessitates significant internal adjustment of the Ordovician timescale.
The code utilised in this study is written by Christoper Brenhin Keller and can be accessed at https://doi.org/10.17605/osf.io/TQX3F (Keller, 2018).
Exact code lines utilised in this study containing the data can be found at https://doi.org/10.26037/yareta:54zokxodvrhlthtwk26htfeyla (Paul, 2024).
The data utilised in this study are taken from Liao et al. (2020) and Lindskog et al. (2017), with details given in the reference list, and all data utilised are available in Supplement Table S1. The original data tables and processed data are available at https://doi.org/10.26037/yareta:54zokxodvrhlthtwk26htfeyla (Paul, 2024).
The supplement related to this article is available online at: https://doi.org/10.5194/gchron-6-325-2024-supplement.
Conceptualisation: ANP, AL, and US. Data curation and formal analysis: ANP. Funding acquisition: AL and US. Investigation: ANP, AL, and US. Visualisation: ANP and AL. Writing (original draft preparation): ANP. Writing (review and editing): ANP, AL, and US.
The contact author has declared that none of the authors has any competing interests.
Publisher's note: Copernicus Publications remains neutral with regard to jurisdictional claims made in the text, published maps, institutional affiliations, or any other geographical representation in this paper. While Copernicus Publications makes every effort to include appropriate place names, the final responsibility lies with the authors.
Anders Lindskog acknowledges funding from the Birgit and Hellmuth Hertz Foundation and the Royal Physiographic Society of Lund. This study benefitted from the SNFNS grant CRSII5_180253 awarded to Urs Schaltegger. We thank the three anonymous reviewers for their constructive comments that helped to improve the paper. We thank associate editor Sandra Kamo and editor Klaus Mezger for handling the paper and providing constructive comments that improved its quality.
This research has been supported by the Schweizerischer Nationalfonds zur Förderung der Wissenschaftlichen Forschung (grant no. CRSII5_180253).
This paper was edited by Sandra Kamo and reviewed by three anonymous referees.
Ahlberg, P., Calner, M., Lehnert, O., Wickström, L., and Lindskog, A.: Regional geology of Västergötland Province, Sweden, in: Field guide for the ISOS 14 post-conference excursion. Geologiska Föreningen Specialpublikation, 9–12, 2023.
Ballo, E. G., Augland, L. E., Hammer, Ø., and Svensen, H. H.: A new age model for the Ordovician (Sandbian) K-bentonites in Oslo, Norway, Palaeogeogr. Palaeocl., 520, 203–213, https://doi.org/10.1016/J.PALAEO.2019.01.016, 2019.
Bergström, S. M.: Use of graphic correlation for assessing event-stratigraphic significance and trans-Atlantic relationships of Ordovician K-bentonites, Proc. Acad. Sci. Est. SSR, 38, 55–59, 1989.
Bowring, J. F., McLean, N. M., and Bowring, S. A.: Engineering cyber infrastructure for U-Pb geochronology: Tripoli and U-Pb-Redux, Geochem. Geophys. Geosyst., 12, Q0AA19, https://doi.org/10.1029/2010GC003479, 2012.
Brenhin Keller, C., Boehnke, P., Schoene, B., and Harrison, T. M.: Stepwise chemical abrasion–isotope dilution–thermal ionization mass spectrometry with trace element analysis of microfractured Hadean zircon, Geochronology, 1, 85–97, https://doi.org/10.5194/gchron-1-85-2019, 2019.
Caricchi, L., Simpson, G., and Schaltegger, U.: Estimates of volume and magma input in crustal magmatic systems from zircon geochronology: The effect of modeling assumptions and system variables, Front. Earth Sci., 4, 48, https://doi.org/10.3389/feart.2016.00048, 2016.
Condon, D. J., Schoene, B., McLean, N. M., Bowring, S. A., and Parrish, R. R.: Metrology and traceability of U-Pb isotope dilution geochronology (EARTHTIME Tracer Calibration Part I), Geochim. Cosmochim. Ac., 164, 464–480, https://doi.org/10.1016/j.gca.2015.05.026, 2015.
Condon, D., Schoene, B., Schmitz, M., Schaltegger, U., Ickert, R. B., Amelin, Y., Augland, L. E., Chamberlain, K. R., Coleman, D. S., Connelly, J. N., Corfu, F., Crowley, J. L., Davies, J. H. F. L., Denyszyn, S. W., Eddy, M. P., Gaynor, S. P., Heaman, L. M., Huyskens, M. H., Kamo, S., Kasbohm, J., Keller, C. B., MacLennan, S. A., McLean, N. M., Noble, S., Ovtcharova, M., Paul, A., Ramezani, J., Rioux, M., Sahy, D., Scoates, J. S., Szymanowski, D., Tapster, S., Tichomirowa, M., Wall, C. J., Wotzlaw, J.-F., Yang, C., and Yin, Q.-Z.: Recommendations for the reporting and interpretation of isotope dilution U-Pb geochronological information, GSA Bull., https://doi.org/10.1130/B37321.1, online first, 2024.
Gerstenberger, H. and Haase, G.: A highly effective emitter substance for mass spectrometric Pb isotope ratio determinations, Chem. Geol., 136, 309–312, https://doi.org/10.1016/S0009-2541(96)00033-2, 1997.
Goldman, D., Sadler, P. M., Leslie, S. A., Agterberg, F. P., and Gradstein, F. M.: The Ordovician Period, in: Geologic Time Scale 2020, edited by: Gradstein, F. M., Ogg, J. G., Schmitz, M. D., and Ogg, G. M., Elsevier, 631–694, https://doi.org/10.1016/C2020-1-02369-3, 2020.
Heck, P. R., Schmitz, B., Baur, H., and Wieler, R.: Noble gases in fossil micrometeorites and meteorites from 470 Myr old sediments from southern Sweden, and new evidence for the L-chondrite parent body breakup event, Meteor. Planet. Sci., 43, 517–528, 2008.
Huyskens, M. H., Zink, S., and Amelin, Y.: Evaluation of temperature-time conditions for the chemical abrasion treatment of single zircons for U-Pb geochronology, Chem. Geol., 438, 25–35, https://doi.org/10.1016/J.CHEMGEO.2016.05.013, 2016.
Jaffey, A. H., Flynn, K. F., Glendenin, L. E., Bentley, W. C., and Essling, A. M.: Precision Measurement of Half-Lives and Specific Activities of 235U and 238U, Phys. Rev. C, 4, 1889, https://doi.org/10.1103/PhysRevC.4.1889, 1971.
Keller, C. B.: Chron.jl: A Bayesian framework for integrated eruption age and age-depth modelling, OSF [code], https://doi.org/10.17605/osf.io/TQX, 2018.
Keller, C. B., Schoene, B., and Samperton, K. M.: A stochastic sampling approach to zircon eruption age interpretation, Geochemical Perspect. Lett., 8, 31–35, https://doi.org/10.7185/GEOCHEMLET.1826, 2018.
Korochantseva, E. V., Trieloff, M., Lorenz, C. A., Buykin, A. I., Ivanonva, M. A., Schwarz, W. H., Hopp, J., and Jessberger, E. K.: L-chondrite asteroid breakup tied to Ordovician meteorite shower by multiple isochron 40Ar-39Ar dating, Meteorit. Planet. Sci., 42, 113–130, https://doi.org/10.1111/j.1945-5100.2007.tb00221.x, 2007.
Liao, S., Huyskens, M. H., Yin, Q.-Z., and Schmitz, B.: Absolute dating of the L-chondrite parent body breakup with high-precision U–Pb zircon geochronology from Ordovician limestone, Earth Planet. Sc. Lett., 547, 116442, https://doi.org/10.1016/j.epsl.2020.116442, 2020.
Lindskog, A. and Eriksson, M. E.: Megascopic processes reflected in the microscopic realm: sedimentary and biotic dynamics of the Middle Ordovician “orthoceratite limestone” at Kinnekulle, Sweden, GFF, 139, 163–183, https://doi.org/10.1080/11035897.2017.1291538, 2017.
Lindskog, A., Costa, M. M., Rasmussen, C. M., Connelly, J. N., and Eriksson, M. E.: Refined Ordovician timescale reveals no link between asteroid breakup and biodiversification, Nat. Commun., 81, 1–8, https://doi.org/10.1038/ncomms14066, 2017.
Lindskog, A., Young, S. A., Bowman, C. N., Kozik, N. P., Newby, S. M., Eriksson, M. E., Pettersson, J., Molin, E., and Owens, J. D.: Oxygenation of the Baltoscandian shelf linked to Ordovician biodiversification, Nat. Geosci., 16, 1047–1053, 2023.
Lindstrom, M.: Volcanic contribution to Ordovician pelagic sediments, J. Sediment. Res., 44, 287–291, https://doi.org/10.1306/74D72A13-2B21-11D7-8648000102C1865D, 1974.
Mattinson, J. M.: Zircon U–Pb chemical abrasion (“CA-TIMS”) method: Combined annealing and multi-step partial dissolution analysis for improved precision and accuracy of zircon ages, Chem. Geol., 220, 47–66, https://doi.org/10.1016/J.CHEMGEO.2005.03.011, 2005.
McKanna, A. J., Koran, I., Schoene, B., and Ketcham, R. A.: Chemical abrasion: the mechanics of zircon dissolution, Geochronology, 5, 127–151, https://doi.org/10.5194/gchron-5-127-2023, 2023.
McKanna, A. J., Schoene, B., and Szymanowski, D.: Geochronological and geochemical effects of zircon chemical abrasion: insights from single-crystal stepwise dissolution experiments, Geochronology, 6, 1–20, https://doi.org/10.5194/gchron-6-1-2024, 2024.
McLaughlin, P. I., Normore, L., Sell, B. K., and Ramezani, J.: Ordovician tephra distribution, tephrochronology and geochronology, Geol. Soc. London, Spec. Publ., 532, 79–90, https://doi.org/10.1144/SP532-2022-267, 2023.
McLean, N. M., Bowring, J. F., and Bowring, S. A.: An algorithm for U-Pb isotope dilution data reduction and uncertainty propagation, Geochem. Geophys. Geosyst., 12, Q0AA18, https://doi.org/10.1029/2010GC003478, 2011.
McLean, N. M., Condon, D. J., Schoene, B., and Bowring, S. A.: Evaluating uncertainties in the calibration of isotopic reference materials and multi-element isotopic tracers (EARTHTIME Tracer Calibration Part II) – ScienceDirect, Geochim. Cosmochim. Ac., 164, 481–501, 2015.
Mezger, K. and Krogstad, E. J.: Interpretation of discordant U-Pb zircon ages: An evaluation, J. Metamorph. Geol., 15, 127–140, https://doi.org/10.1111/J.1525-1314.1997.00008.X, 1997.
Nielsen, A. T., Ove, J., Ebbestad, R., Hammer, Ø., Alexander, D., Harper, T., Lindskog, A., Mac, C., Rasmussen, Ø., and Stouge, S.: The Ordovician of Scandinavia: a revised regional stage classification, Geol. Soc. London, Spec. Publ., 532, 267–315, https://doi.org/10.1144/SP532-2022-157, 2023.
Paul, A. N.: Repository For: Short Communication: Resolving the Discrepancy between U–Pb Age Estimates for the “Likhall” Bed, a Key Level in the Ordovician Timescale, Yareta [data set], https://doi.org/10.26037/yareta:54zokxodvrhlthtwk26htfeyla, 2024.
Rasmussen, J. A., Thibault, N., and Rasmussen, C. M. Ø.: Middle Ordovician astrochronology decouples asteroid breakup from glacially-induced biotic radiations, Nat. Commun., 12, 6430, https://doi.org/10.1038/s41467-021-26396-4, 2021.
Samperton, K. M., Schoene, B., Cottle, J. M., Brenhin Keller, C., Crowley, J. L., and Schmitz, M. D.: Magma emplacement, differentiation and cooling in the middle crust: Integrated zircon geochronological–geochemical constraints from the Bergell Intrusion, Central Alps, Chem. Geol., 417, 322–340, https://doi.org/10.1016/J.CHEMGEO.2015.10.024, 2015.
Schaltegger, U., Ovtcharova, M., Gaynor, S. P., Schoene, B., Wotzlaw, J.-F., Davies, J. F. H. L., Farina, F., Greber, N. D., Szymanowski, D., and Chelle-Michou, C.: Long-term repeatability and interlaboratory reproducibility of high-precision ID-TIMS U–Pb geochronology, J. Anal. At. Spectrom., 36, 1466–1477, https://doi.org/10.1039/D1JA00116G, 2021.
Schmitz, B., Harper, D. A. T., Peucker-Ehrenbrink, B., Stouge, S., Alwmark, C., Cronholm, A., Bergström, S. M., Tassinari, M., and Xiaofeng, W.: Asteroid breakup linked to the Great Ordovician Biodiversification Event, Nat. Geosci., 1, 49–53, 2008.
Schmitz, B., Farley, K. A., Goderis, S., Heck, P. R., Bergström, S. M., Boschi, S., Claeys, P., Debaille, V., Dronov, A., van Ginneken, M., Harper, D. A. T., Iqbal, F., Friberg, J., Liao, S., Martin, E., Meier, M. M. M., Peucker-Ehrenbrink, B., Soens, B., Wieler, R., and Terfelt, F.: An extraterrestrial trigger for the mid-Ordovician ice age: Dust from the breakup of the L-chondrite parent body, Sci. Adv., 5, eaax4184, https://doi.org/10.1126/sciadv.aax4184, 2019.
Schmitz, M. D. and Schoene, B.: Derivation of isotope ratios, errors, and error correlations for U-Pb geochronology using 205Pb-235U-(233U)-spiked isotope dilution thermal ionization mass spectrometric data, Geochem. Geophys. Geosyst., 8, Q08006, https://doi.org/10.1029/2006GC001492, 2007.
Schoene, B., Crowley, J. L., Condon, D. J., Schmitz, M. D., and Bowring, S. A.: Reassessing the uranium decay constants for geochronology using ID-TIMS U-Pb data, Geochim. Cosmochim. Ac., 70, 426–445, https://doi.org/10.1016/j.gca.2005.09.007, 2006.
Servais, T. and Harper, D. A. T.: The Great Ordovician Biodiversification Event (GOBE): definition, concept and duration, Lethaia, 51, 151–164, https://doi.org/10.1111/LET.12259, 2018.
Trayler, R. B., Meyers, S. R., Sageman, B. B., and Schmitz, M. D.: Bayesian integration of astrochronology and radioisotope geochronology, Geochronology, 6, 107–123, https://doi.org/10.5194/gchron-6-107-2024, 2024.
von Quadt, A., Wotzlaw, J.-F., Buret, Y., Large, E. S. J., Peytcheva, I., and Trinquier, A.: High-precision zircon U/Pb geochronology by ID-TIMS using new 1013 ohm resistors, J. Anal. At. Spectrom., 31, 658–665, https://doi.org/10.1039/C5JA00457H, 2016.
Watson, E. B., Cherniak, D. J., Hanchar, J. M., Harrison, T. M., and Wark, D. A.: The incorporation of Pb into zircon, Chem. Geol., 141, 19–31, 1997.
Weber, G., Caricchi, L., Arce, J. L., and Schmitt, A. K.: Determining the current size and state of subvolcanic magma reservoirs, Nat. Commun., 111, 1–14, https://doi.org/10.1038/s41467-020-19084-2, 2020.
Widmann, P., Davies, J. H. F. L., and Schaltegger, U.: Calibrating chemical abrasion: Its effects on zircon crystal structure, chemical composition and UPb age, Chem. Geol., 511, 1–10, https://doi.org/10.1016/J.CHEMGEO.2019.02.026, 2019.