the Creative Commons Attribution 4.0 License.
the Creative Commons Attribution 4.0 License.
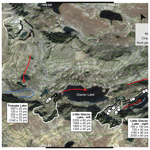
10Be age control of glaciation in the Beartooth Mountains, USA, from the latest Pleistocene through the Holocene
Elizabeth G. Ceperley
Claire Vavrus
Shaun A. Marcott
Jeremy D. Shakun
Marc W. Caffee
Alpine glaciers in the western United States are often associated with late Holocene Little Ice Age (LIA) advances. Yet, recent studies have shown many of these glacial landforms are remnants of latest Pleistocene retreat with only the most cirque-proximal moraines preserving LIA activity. Additionally, the timing and magnitude of glacial advances during the Neoglacial–LIA interval remains uncertain, with presumed maximum extents occurring during the LIA driven by lower Northern Hemisphere insolation levels. Here we present 10Be surface exposure ages from a glacial valley in the Beartooth Mountains of Montana and Wyoming, United States. These new data constrain the presence of the glacier within 2–3 km of the cirque headwalls by the end of the Pleistocene with implications for large-scale retreat after the Last Glacial Maximum. Cirque moraines from two glaciers within the valley preserve a late Holocene readvance, with one reaching its maximum prior to 2.1±0.2 ka and the other 0.2±0.1 ka. Age variability among the moraines demonstrates that not all glaciers were at their largest during the LIA and presents the possibility of regional climate dynamics controlling glacial mass balance.
- Article
(8417 KB) - Full-text XML
- BibTeX
- EndNote
Glacier retreat is one of the clearest indicators of the cryosphere's response to recent global warming. Photographic and satellite imagery of reductions in global glacier extent from the past century document widespread retreat (Bolch, 2007; Catania et al., 2018). Within the last 2 decades alone, the rate of ice loss from mountain glaciers has doubled (Hugonnet et al., 2021). However, analysis of glacier sensitivity to climate change using the instrumental record is limited by data which only goes back decades (Braumann et al., 2020). We are therefore reliant on geologic records of past glacier activity to determine the influence of anthropogenic warming on glacier mass balance relative to natural variability. In the early Holocene, glaciers within the western United States (US) and Canada were at minimum lengths, similar to modern values, with peak Northern Hemisphere (NH) summer insolation values contributing to the retreat (Solomina et al., 2015). Reactivation of glaciation after 6 ka (“Neoglacial”) occurred as glaciers advanced to their greatest Holocene extent (Porter and Denton, 1967; Solomina et al., 2016, 2015). Relative to the last glacial period, the Holocene was considerably more stable in terms of its climate variability. However, variable timing of glacier maximum extent throughout the Neoglacial suggests other mechanisms controlled glacier size besides NH insolation. Well-dated records of late Holocene glaciation are therefore required to accurately assess forcing mechanisms for the Neoglacial.
Many mountain ranges of the western US remained below the southern reaches of the Laurentide Ice Sheet during the Last Glacial Maximum (LGM, 26–19 ka; Clark et al., 2009) where numerous alpine glaciers and ice caps nucleated (Laabs et al., 2020). LGM glacial positions are recorded as down-valley moraines extending as much as 50 km from the cirques while younger, high-elevation glacial landforms are preserved within 1–2 km of the headwall (Davis, 1988; Davis et al., 2009). These younger glacial deposits were originally thought to record Neoglaciation, but recent dating concluded that many of these landforms across the western US are in fact remnants of latest Pleistocene and earliest Holocene glaciation (Marcott et al., 2019). The questions then arise: what is the record of late Holocene glaciation in the western US, if any exists at all? What does it tell us about the response of mountain glaciers to climatic forcings throughout the Holocene, and were they sufficient to permit glacial regrowth?
Here we present 10Be exposure ages from high-elevation glacial landforms in the Beartooth Mountains of Montana (MT) and Wyoming (WY) to determine the timing of glaciation in this sector of the western US. Together with previously published ages on downvalley LGM moraines, this new chronology sheds light on the rate and magnitude of glacier retreat during the last deglaciation and potential Neoglacial regrowth during the late Holocene.
The Beartooth Mountains extend from southwestern MT into northern WY and are a broadly arcuate mountain range reaching ∼30 km at their widest. At their base, the Mill Creek–Stillwater Fault Zone separates the northern foothills of the Beartooth Mountains from the Great Plains (Fig. 1; Bevan, 1923; Montgomery and Lytwyn, 1984) with ∼1800 m relief between the plains (∼1800 m a.s.l.) and the highest elevations in the mountain range (>3600 m a.s.l.). High plateaus have been dissected by fluvial and glacial erosion. Numerous cirques and northward-oriented glacial valleys are present within the Beartooths, whereas the Absaroka Range to the south exhibits more southward- and eastward-oriented glacial valleys. The eastern portion of the mountain range is predominantly Archean quartz-rich granitic gneisses and migmatites with inclusions of metasedimentary and meta-igneous rocks (Van Gosen et al., 2000). Coarse crystalline pegmatitic dikes are common throughout the region (Bevan, 1923).
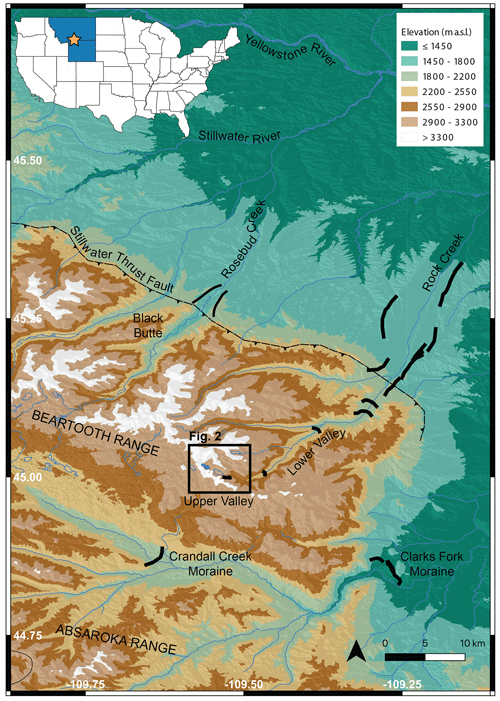
Figure 1Regional map of the Beartooth Mountains in Montana and Wyoming, United States. Brown colors indicate higher elevation, and green colors indicate lower elevation. Solid black lines show the location of prominent moraines including those mapped by Graf (1971) in the Rock Creek drainage. The inset map shows the location of Montana (MT) and Wyoming (WY) highlighted in blue, with the approximate location of our study area indicated by the star. Elevation data from the U.S. Geological Survey 3D Elevation Program (U.S. Geological Survey, 20171130, USGS 13 arc-second n46w110 1 x 1 degree: U.S. Geological Survey).
Glacial landforms in the mountain range record multiple phases of glaciation, including the Bull Lake (Marine Isotope Stage, MIS, 6) and Pinedale (MIS 2) glaciations. Bull Lake glaciation within the northeast sector of the Beartooths is recorded as remnant outwash terraces of higher elevation to those of the younger Pinedale terraces (Ballard, 1976). Morainal evidence, however, is sparse for Bull Lake glacier limits and is suggestive of similar, or lesser, glacial extents to Pinedale advances (Licciardi and Pierce, 2018). During the last glaciation, high-elevation ice flowed southward to the Yellowstone Plateau where it coalesced with similar glaciers from the surrounding mountains forming the Yellowstone ice cap, which acted as an ice divide at the LGM (Licciardi and Pierce, 2018, 2008). Numerous long (>20 km) glacial valleys are present along the periphery of the Greater Yellowstone Glacial System (GYGS), including those of the northern Beartooths. Glacial deposits including erratics, moraines, and outwash terraces are common within these valleys and provide abundant geologic evidence of past glacial activity from the LGM and deglaciation. Higher in the valleys, proximal to cirque headwalls, additional glacial evidence is preserved as sharp-crested moraines, rock glaciers, and protalus lobes (Davis, 1988; Graf, 1971). Most features are mapped within 1–2 km from cirque headwalls and may represent multiple phases of glaciation (Davis, 1988). Based on geomorphologic characteristics, Graf (1971) identified such features in the Beartooths as two periods of late Holocene glaciation. However, geochronological data are limited by relative-age methods and their associated uncertainties (Davis, 1988).
The Rock Creek drainage, a ∼25 km long glacial valley, exits from the northeast corner of the Beartooth Range with associated cirque valleys, including Glacier Lake Valley (study area), located along the border between MT and WY and just north of Yellowstone National Park (Figs. 1 and 2). The lower portion of this drainage (herein referred to as the Lower Valley) is fed by five higher-elevation glacial cirques, trends southwest–northeast, and drains into the town of Red Lodge, MT. Sets of lateral moraines extend north from the valley mouth and bound the town on the eastern and western sides. The lateral moraines define an elongate and relatively narrow former glacier, and they are similar in morphology and size to moraines in valleys 25 km to the west beneath Black Butte. Outboard of the moraines, there are fluvially modified hills unaffected by glacial activity, while outwash sediments between the lateral moraines suggest extensive meltwater drainage from the Lower Valley. A terminal moraine for this glacier is not preserved, likely due to erosion from meltwater flowing north to the Yellowstone River (Fig. 1). Graf (1971) mapped five moraines within the Lower Valley, although preservation is patchy, including stream breaches ranging from 70 m wide to as wide as the valley floor in some cases. Steep sloped, rocky walls define the valley width, which averages 1.5 to 2.0 km at its widest and narrows further up the valley. Occasional large (>1 m) boulders along the valley floor exhibit glacially faceted surfaces and striations. The Lower Valley is gently sloped, gaining only 800 m of elevation from the Great Plains over 25 km.
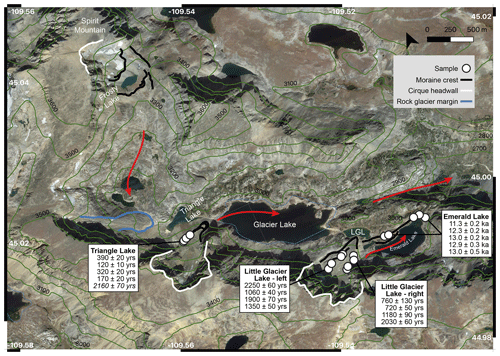
Figure 2Glacier Lake Valley map highlighting the names of important locations discussed in the paper. White lines indicate the cirque headwalls. Solid black lines show the location of moraines. White circles indicate the locations of surface exposure samples with their ages contained in the respective boxes. Statistically identified outliers are italicized. LGL stands for Little Glacier Lake. Red arrows indicate generalized ice flow direction. Elevation data are from the U.S. Geological Survey 3D Elevation Program (U.S. Geological Survey, 20171130, USGS 13 arc-second n46w110 1 x 1 degree: U.S. Geological Survey).
There is a steep, 400 m high transition from the Lower Valley to Glacier Lake valley (herein referred to as the Upper Valley), capped by an exposed, glacially abraded, bedrock lip. Four lakes are located between 2960 and 2970 m a.s.l. within the Upper Valley (from east to west): Emerald Lake, Little Glacier Lake, Glacier Lake, and Triangle Lake (Fig. 2). Two of the lakes, Emerald Lake and Little Glacier Lake, are bounded by the bedrock lip to the north. To the south, Emerald Lake is bounded by an exposed bedrock cliff, while Little Glacier Lake is bounded by a bouldery moraine. A curved boulder-covered moraine exists in the gentle topography between the two lakes (referred to as the Emerald Lake moraine) and is the stratigraphically oldest moraine in the Upper Valley (Graf, 1971). The Emerald Lake moraine has a subdued cross-profile suggestive of moraine degradation over time from the removal of finer-grained material from the peak to the flanks (Putkonen et al., 2008; Sortor, 2022). The location and size of Glacier Lake is controlled by steep rocky walls to the north and south with colluvial fans along the base of the southern wall. Two bedrock knobs separate Glacier Lake from Triangle Lake to the west, with morainal deposits restricting water flow between the two (Graf, 1971). To the northwest of Triangle Lake, a rounded and smoothed bedrock exposure increases elevation as the valley changes orientation to north–south and gains another ∼450 m of elevation. A small cirque below the peak of Spirit Mountain (3744 m a.s.l.) contains a lake, Frosty Lake, and two moraines along both the down- and up-valley shores. The down-valley moraine is low-relief, bouldery, and rests on the cirque lip. The up-valley moraine is 50 m tall, sharp-crested, within 25 m of modern ice, and morphologically similar to the moraine at Triangle Lake. No prominent moraines are present between Triangle Lake and those at Frosty Lake.
The cirque near Little Glacier Lake preserves two moraines with an additional moraine in the cirque near Triangle Lake (Fig. 2). The moraines around Little Glacier Lake have high-angle lateral slopes of large (>1 m) angular boulders mixed with coarse to fine sands and all grain sizes in between (Fig. 3). The toe of the moraine is lower relief (15 m), dominated by boulders, and rests along the southern shore of Little Glacier Lake. The left-lateral moraine rests on a bedrock outcrop at 3022 m a.s.l. (Fig. 3b). Ribbed crests of boulder deposits are found inboard of the left-lateral moraine and maintain a similar elevation throughout. Between the lateral moraines, the surface elevation remains fairly consistent, with drastic changes in slope only along the distal sides of the moraines. While the moraine crests are easily defined, abundant boulder debris coverage within the moraine limits is suggestive of rock glacier activity or rockfall from over-steepened cliff faces. Small patches of modern ice are visible near the headwall but are covered in debris further downslope.
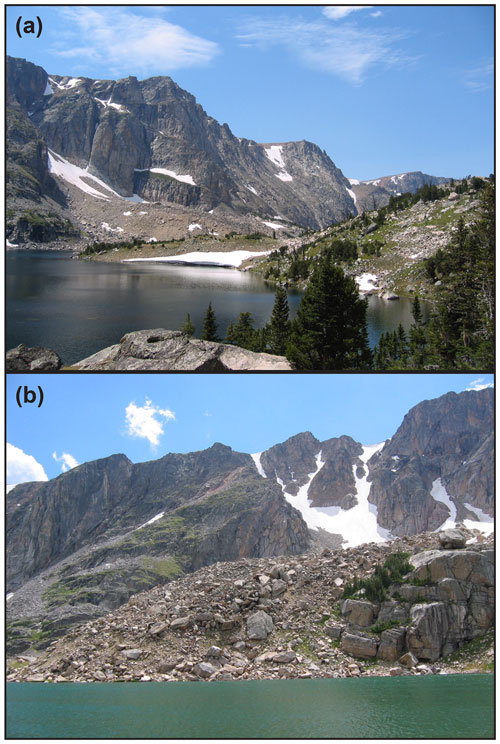
Figure 3Beartooth moraines: (a) Emerald Lake moraine on the left, with the Little Glacier Lake moraines in the distance. (b) Toe and left-lateral aspect of the Little Glacier Lake moraine and bedrock knob.
A steep-sloped moraine is found along the southern shore of Triangle Lake, ∼1.2 km north of a cirque headwall containing a small (∼0.1 km2) debris-covered glacier. The moraine increases in relief from 35 m near the terminus to 80 m along the lateral aspects and contains abundant coarse sands and gravels among numerous angular boulders (>0.4 m in height). The peak of the moraine is narrow and exhibits minimal deflation, yet occasional patches of grass and soil suggest the surficial deposits are not recent. From the terminus, the moraine curves to the east to intersect a near-vertical cliff face and is covered with boulders, some up to ∼10 m in size. The western aspect of the moraine gains 130 m elevation before encountering a glacially smoothed bedrock knob. Above the bedrock knob, the moraine continues another 60 m before reaching the glacier.
To determine the timing of glaciation within Upper Valley, we sampled glacially deposited boulders for cosmogenic 10Be surface exposure dating from four moraines: one at Triangle Lake, two at Little Glacier Lake, and one at Emerald Lake moraine (Fig. 4). Samples were collected over two field seasons in 2006 (Emerald Lake and Little Glacier Lake) and 2017 (Triangle Lake and Little Glacier Lake) using hammer and tungsten carbide-tipped chisels. Strict sample selection criteria were followed to maximize the accuracy of nuclide accumulation representative of initial deposition by the glacier (Dunai, 2010; Gosse and Phillips, 2001). Boulders selected for sampling exhibited no signs of surficial erosion or pitting that would remove accumulated nuclides. Samples were limited to within the top 5 cm from flat-topped boulders to ensure the highest rates of in situ nuclide production. All samples were collected on or near the mapped moraine crest to minimize potential post-depositional movement and associated reduction of nuclide accumulation on the sampled surface. Each boulder was a minimum of 0.4 m in height with an average boulder height of 1.5 m. Potential boulder burial or exhumation is unlikely given boulder height and morphological characteristics of the moraines. Both the Little Glacier Lake and Triangle Lake moraines exhibit little to no soil cover, which suggests exhumation of boulders from previous cover is unlikely. Latitude, longitude, and elevation were recorded for each sample using a handheld GPS unit and verified using high-resolution DEMs and topographic maps. Surface inclination of each sample was measured using a Brunton compass, and topographic shielding was recorded in the field using a clinometer. Shielding scaling factors for each sample were calculated using the CRONUS online Topographic Shielding Calculator (http://stoneage.ice-d.org/math/skyline/skyline_in.html, last access: 14 July 2018; Balco et al., 2008).
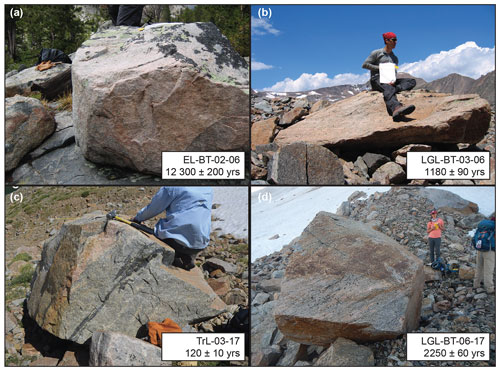
Figure 4Sample boulders for 10Be surface exposure dating discussed in this project. (a) EL stands for Emerald Lake. (b, d) LGL stands for Little Glacier Lake. (c) TrL stands for Triangle Lake. The 1σ analytic uncertainty shown for each age.
A total of 18 samples were collected and processed for 10Be extraction that best represent our selection criteria: five boulders from Emerald Lake, four from the inner Little Glacier Lake moraine, four from the outer Little Glacier Lake moraine, and five from Triangle Lake.
Boulder samples were processed for 10Be extraction at the University of Wisconsin – Madison. The sample processing procedures are adapted from laboratories at Oregon State University, the University of Vermont Cosmogenic Nuclide Laboratory, and the University of New Hampshire (Corbett et al., 2016; Kohl and Nishiizumi, 1992; Licciardi, 2000; Marcott, 2011). Each rock sample was sawed to isolate the top 2–5 cm, crushed and sieved to a 250–710 µm size fraction, magnetically separated, and etched in concentrated HCl and dilute 2 % acid solutions. A minimum of three rounds of etching were completed for each sample. Chemical frothing was performed on all non-magnetic grains in order to isolate quartz from feldspar. The remaining quartz grains were isolated using additional etches. Quartz purity was measured by inductively coupled plasma optical emission spectroscopy (ICP-OES) at the University of Colorado Boulder Department of Geological Sciences and the University of Wisconsin – Madison Water Science and Engineering Laboratory.
The chemically etched and pure quartz were dissolved in concentrated HF, after an addition of 9Be carrier solution prepared from raw beryl (OSU White standard; 9Be concentration of 251.6±0.9 ppm) (Marcott, 2011). We used anion and cation exchange chromatography to separate Fe, Ti, and Al from Be, and BeOH was precipitated in a pH 8 solution. BeOH gels were converted to BeO by heating to 900–1000 ∘C with a rapid incinerator, mixed with Nb powder in quartz crucibles, and packed into stainless steel cathodes for accelerator mass spectrometry (AMS) analysis.
All ratios were measured at the Purdue University Rare Isotope Measurement Laboratory (PRIME Lab) and normalized to standard 07KNSTD3110, which has an assumed ratio of 2.85×1012 (Nishiizumi et al., 2007). Sample ratios were corrected using batch-specific blank values that ranged from to (n=3; Table 1). 10Be concentrations presented in Table 1 are corrected from process blanks.
Table 1Sample data and 10Be concentrations.
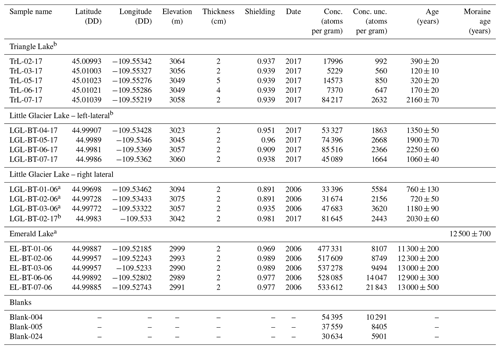
a Samples that used Blank-024 in calculations.
b Samples that used Blank-004 and Blank-005 in calculations.
Exposure ages are calculated using version 3.0 of the original online calculator as described in Balco et al. (2008; herein referred to as version 3.0) and the time-dependent scaling scheme of Lifton et al. (2014; LDSn). We use the 10Be production rate in quartz as determined from Promontory Point, UT (PPT; Lifton et al., 2015), based on proximity to the study site (∼700 km) and the high confidence in the quality of constraining samples. We note that a recent review of western US surface exposure ages follows similar methods for recalculating previous published data (Laabs et al., 2020; Licciardi and Pierce, 2018) including those for the GYGS. Wide-ranging rates of surface erosion experienced by glacial erratics are suggested in the literature with rates within the order of 0.0 to 0.1 cm ka−1 (Gillespie and Bierman, 1995). Based on the presumed young age of our sampled deposits, we assume surface erosion of the boulders was negligible and therefore do not account for it in our calculations. We follow the procedures outlined in Laabs et al. (2020) and assume the effects of snow cover to be negligible. Individual exposure ages are reported as years before collection date (per Version 3.0 calculations) and with 1σ analytical uncertainty. Moraine ages are reported as the arithmetic mean and standard deviation of the boulder ages to present a conservative uncertainty estimate. We note that distribution ages reported using error-weighted mean and uncertainty tend to favor younger exposure ages with lower analytical uncertainty, which are more likely to be influenced by geological processes (e.g., surface erosion, post-depositional movement), leading to erroneously young ages (Balco, 2011; Laabs et al., 2020; Licciardi and Pierce, 2008). Outliers are identified using Chauvenet's criterion and interquartile range tests.
Resulting 10Be exposure ages demonstrate glaciation within the Upper Valley from the latest Pleistocene and the late Holocene with all ages in stratigraphic order. Five exposure ages from the stratigraphically oldest moraine at Emerald Lake range from 11.3±0.2 to 13.0±0.5 ka (Table 1). Together these samples have a mean age and standard deviation of 12.5±0.7 ka. We note that sample EL-BT-01-06 (11.3±0.2 ka) is 0.9 ka younger than the next youngest sample (EL-BT-02-06; 12.3±0.2 ka), but it cannot be rejected using either of the statistical tests. Removing sample EL-BT-01-06 from the population increases the mean age to 12.8 ka, which falls within 1σ uncertainty of our reported age and therefore does not change our interpretations.
Samples (n=4) from the Little Glacier Lake left-lateral moraine yield exposure ages ranging from 1060±40 to 2250±60 years (Table 1). The Little Glacier Lake right-lateral moraine yielded four exposure ages ranging from 720±50 to 2030±60 years (Table 1). A ∼1300-year range exists between the oldest and youngest age of the population; however, no samples are rejected using the statistical tests described. The samples in both populations were likely affected by geologic processes.
Five samples from the moraine near Triangle Lake yield exposure ages ranging from 120±10 to 2160±70 years. Sample TrL-07-17 (2160±70 years) is identified as an outlier using both previously mentioned statistical tests. The four remaining samples exhibit an approximate bimodal distribution but overlap within 2σ uncertainty. In our discussion, we interpret the remaining age range of 120±10 to 390±20 years.
5.1 Variable rates of western US glacier retreat from the LGM to the Younger Dryas
During the last deglaciation, the timing and rate of retreat of western US glaciers varied spatially (Laabs et al., 2020). Broadly, glacial retreat from LGM margins in the western US began between 22 and 18 ka (Shakun et al., 2015; Young et al., 2011). Glaciers from the northern GYGS fall within this window of deglacial onset, with two terminal moraines yielding 10Be exposure ages of 19.8±0.9 and 18.2±1.3 ka (Fig. 5; Licciardi and Pierce, 2018). While no geochronological control exists for the lateral moraines near Red Lodge, a lack of moraines outboard of them suggests they represent a terminal position of the glacier. They are also morphologically similar to other LGM-mapped moraines within the Beartooths (Black Butte; 25 km west of Red Lodge) and are proximal to the dated moraines at Clarks Fork (19.8±0.9 ka) and Pine Creek (18.2±1.3 ka) that are part of the same regional glacial system. Therefore, for our purposes, we assume the age and therefore the onset of deglaciation of the Red Lodge moraines to fall within the time window of 22 to 18 ka.
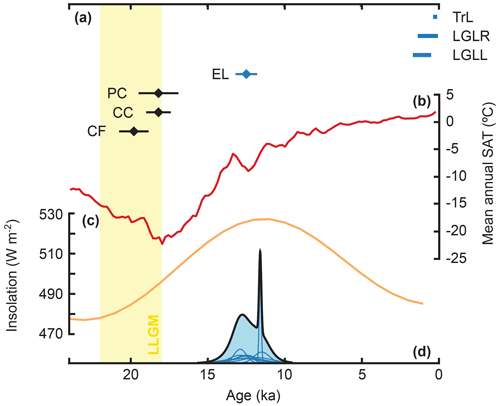
Figure 5LGM, Deglacial, and Holocene periods; diamonds indicate moraine ages. (a) Blue diamonds are ages from this study. Blue bars represent the age range for late-Holocene samples. TrL stands for Triangle Lake, LGLL stands for Little Glacier Lake left-lateral moraine, and LGLO stands for Little Glacier Lake right lateral moraine. Black diamonds are ages from Licciardi and Pierce (2018). PC stands for Pine Creek moraine, CC stands for Crandall Creek, and CF stands for Clarks Fork. (b) The red line is the mean annual surface temperature for our study area taken from Osman et al. (2021). (c) The orange line is mean annual insolation for 45∘ N. (d) Bottom probability distribution shaded in blue is for the compilation of western US Younger Dryas moraines taken from Marcott et al. (2019), Licciardi and Pierce (2018), and the Emerald Lake moraine from this study. The yellow box highlights the timing of the local LGM from 22 to 18 ka.
Rapid retreat of the Clarks Fork glacier to the Crandall Creek moraine between 19.8±0.9 and 18.2±0.8 ka was followed by the construction of multiple recessional moraines as retreat slowed (Licciardi and Pierce, 2018). Initial rapid retreat of the Clarks Fork glacier is hypothesized to have occurred as migration of the Yellowstone Ice Cap to the southwest created a precipitation shadow for the northeast portion of the GYGS, thus affecting glacial mass balance negatively (Licciardi and Pierce, 2018, 2008). Synchronous changes in North American precipitation patterns were affecting glacial mass balance in the central northern region of the US (Lora and Ibarra, 2019). These changes in precipitation include migration of the dominant atmospheric jet stream (Lora et al., 2017; Manabe and Broccoli, 1985) and changes in the seasonality of precipitation delivery to certain regions (Lora and Ibarra, 2019). Slower retreat of the Clarks Fork glacier during the Late Pinedale (16–13 ka) is attributed to a reduction in the precipitation shadow in the northeastern GYGS as the Yellowstone Ice Cap continued its migration to the southwest (Licciardi and Pierce, 2018). After ∼14 ka, a more regionally coherent response of glacier retreat occurred in response to warming from increased atmospheric greenhouse gas concentrations (Marcott et al., 2019, 2014; Shakun et al., 2015).
Graf (1971) mapped five moraines within the Lower Valley between our prescribed LGM moraines and the Upper Valley, which mark the location of the glacier as it retreated from its terminal position (Fig. 1). Based on geomorphological evidence such as distance regression, soil development, and shape index, the two furthest down-valley moraines were associated with Bull Lake glaciation and the three more up-valley moraines as Pinedale terminal moraines. Furthermore, Graf (1971) assigned the Pinedale moraines correlative ages with other western US deposits ranging from 23.0 ka for the outermost to 11.5 ka for a moraine found near the steep transition from Lower Valley to the Upper Valley. However, based on the reasons described above, we argue all deposits within the Lower Valley are more likely to be representative of post-LGM deglaciation similar to proximal glacier valleys within the GYGS (Laabs et al., 2020; Licciardi and Pierce, 2018, 2008). Additionally, the age of glacial retreat we obtained for the Emerald Lake moraine (12.5±0.7 ka) inboard from the Graf (1971) 11.5 ka Lower Valley moraine suggests stabilization of the glacial prior to 12.5±0.7 ka and is therefore in stratigraphic disagreement with this assigned age. As such, the Lower Valley deposits must be older than 12.5±0.7 ka and represent a period of glaciation between the end of the LGM (22 to 18 ka) and formation of the Emerald Lake moraine (Fig. 5).
The five Lower Valley moraines between Red Lodge and the Upper Valley suggest multiple periods of stagnation or glacial readvance during the last deglaciation. A lack of numerical age control limits our ability to assess the rate and timing of retreat within the Lower Valley. However, stratigraphic relationships of the moraines and chronologic control higher in the valley permit a certain amount of book-ending.
The Emerald Lake moraine marks the next geochronologically constrained position of the glacier following the LGM. Using the time window of 22 to 18 ka for the onset of LGM deglaciation in Red Lodge and the age of the Emerald Lake moraine as limiting ages we determined a maximum and minimum mean retreat rate of the glacier of 4.9 m yr−1 (18.0–12.5 ka) and 2.8 m yr−1 (22.0–11.6 ka), respectively. These values are lower than those recorded for the nearby Clarks Fork glacier, which lost 75 %–90 % of its LGM length between 19.8±0.9 and 18.2±0.8 ka at a mean retreat rate of 28.0 m yr−1 (Licciardi and Pierce, 2018).
We hypothesize that retreat of the Rock Creek glacier from its LGM limit began later in the window of LGM retreat (22 to 18 ka) similar to the Pine Creek moraine (18.2±1.3 ka). After which the Rock Creek glacier experienced slower retreat similar to that of the Clarks Fork glacier after abandoning the Crandall Creek moraine (18.2±0.8 ka), thus allowing for the formation of morainal features within the Lower Valley. Eventually the glacier left the Lower Valley in response to rising atmospheric greenhouse gases.
5.2 The Younger Dryas
The presence of the Emerald Lake moraine shows the location of the glacier in the latest Pleistocene, older than the previously proposed Neoglacial age (Graf, 1971), and marks a pinning point of glaciation during retreat from the LGM limits. Additionally, these data contribute to a growing data set of western US moraine ages supporting a regional response of mountain glaciers to the Younger Dryas (YD; Marcott et al., 2019).
The Emerald Lake moraine marks the first location of the glacier margin in the Upper Valley ∼27 km up valley from the LGM position. Presence of the moraine indicates stillstand or readvance of the glacier during the latest Pleistocene, perhaps in response to YD cooling (12.9–11.7 ka; Davis et al., 2009; Gosse et al., 1995). Geochronological studies from glaciers in additional western US mountain ranges identify a similar glacier responses to YD cooling (Gosse et al., 1995; Licciardi and Pierce, 2008; Marcott et al., 2019), while other paleoclimate proxies identify a YD signal in the region (Mix et al., 1999; Praetorius et al., 2020, 2015; Vacco et al., 2005). However, uncertainty in the exposure ages, and differences in production rate calculations, prevent conclusively attributing formation of the Emerald Lake moraine to the early or later portions of the YD stadial. Two potential scenarios arise: (1) deposition of the moraine early in the YD cooling with glacier retreat continuing throughout the stadial and (2) deposition in response to YD cooling and retreat following abrupt warming at the end of the YD.
The age of the Emerald Lake moraine places retreat of the glacier from this location 12.5±0.7 ka with glacial stabilization occurring prior to this time (Fig. 5). Morainal deposits near the Lake Solitude cirque lip in the nearby Teton Range (∼175 km to the south) are dated to 12.9±0.7 ka (Licciardi and Pierce, 2008; recalculated in Licciardi and Pierce, 2018). Together the ages of the Emerald Lake and Lake Solitude moraines imply a glacier response to the YD cooling with retreat during or after the stadial. A compilation of YD-aged deposits from the western US (Marcott et al., 2019), calculated using the PPT production rate and LSDn scaling scheme, highlight nine separate moraine exposure ages (including the Emerald Lake and Lake Solitude moraines) between 12.9±0.7 ka (Licciardi and Pierce, 2008) and 11.5±0.5 ka (Marcott et al., 2019). The mean age and standard deviation of these moraines is 12.3±0.6 ka, while probability density indicates a higher peak earlier in the stadial (12.8 ka). All moraines in the compilation exist between 37 and 45∘ N and above 2765 m elevation. Taken together, these exposure ages suggest a regional glacial response to the YD stadial, with variability in timing of morainal abandonment – potentially driven by differences in precipitation patterns (Lora and Ibarra, 2019), atmospheric lapse rate (Loomis et al., 2017; Zhang et al., 2019), or topographic control (Barr and Spagnolo, 2015). However, multi-centennial-scale uncertainties within each age prevent conclusive attribution of moraines to early or late periods of the YD.
However, the deflated nature of the Emerald Lake moraine lies in contrast to the much younger Triangle Lake and Frosty Lake moraines, as well as to the older LGM moraines found at Clarks Fork and Black Butte. Similar low-profile morainal deposits associated with mountain glaciation are attributed to high-frequency glacier length variability during deglaciation (Barth et al., 2018). Therefore, the subdued profile of the Emerald Lake moraine suggests any period of stagnation was a short-lived one and/or was characterized by low depositional rates. Any forcing of this response had a minor impact on the overall trend of glacier retreat.
5.3 Constraints on Neoglaciation and the Little Ice Age
Ages from the Little Glacier Lake and Triangle Lake moraines indicate Upper Valley glacier activity within the late Holocene (Fig. 6). Glaciation in the western US is suggested to have reached a minimum in the early Holocene due to high Northern Hemisphere summer insolation (Porter and Denton, 1967). Multiple archives across the Northern Hemisphere highlight a readvance of glaciers in the later Holocene (Munroe et al., 2012; Porter and Denton, 1967; Reyes et al., 2006; Solomina et al., 2015) due to declining summer temperatures (Marcott et al., 2013; McKay et al., 2018). Late Holocene, Neoglacial deposits are found in numerous mountain ranges across the western US and Canada, Greenland, Europe, and Asia (Briner and Porter, 2018; Graf, 1971; Marcott et al., 2019; Munroe et al., 2012; Reyes et al., 2006; Solomina et al., 2015).
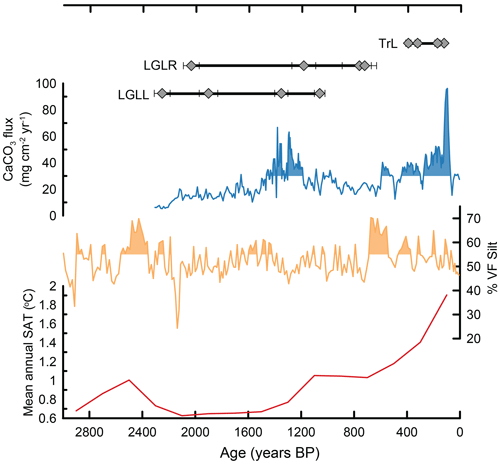
Figure 6(a) Ages for the late Holocene moraines from this study adjusted to reflect years before present. Black lines indicate the age range for all samples within a moraine population. Gray diamonds are individual 10Be ages with 1σ uncertainty. (b) The blue line shows the CaCO3 flux from Harrison Lake in Glacier National Park (Munroe et al., 2012). Shaded areas indicate greater than mean values for the time series and are interpreted as increased glacial activity in the original study. (c) The orange line shows the percent of very fine silt fraction from Cracker Lake in Glacier National Park (Munroe et al., 2012). Shaded areas indicate greater than mean values and increased glacial activity. (d) The red line is the mean annual surface temperature for our study region taken from Osman et al. (2021).
Surface exposure ages from boulders within the Little Glacier Lake moraines exhibit a broad range suggestive of geologic uncertainty. One possible explanation for the wide range of ages is that some boulders contain accumulated nuclides from previous periods of exposure and are perhaps sourced from rock fall from the nearby cirque headwall and cliff faces. Based on geomorphological characteristics, including the bouldery nature and steep toe of the moraine, another possibility is that this moraine is the product of rock glaciation with lower rates of erosion less likely to remove previously accumulated nuclides from past periods of exposure. The sample population as a whole demonstrates a multi-modal distribution, which could be interpreted as numerous periods of glaciation. As such, here we discuss the range of ages and interpret them as a limiting range of deglaciation from the moraine and suggestive of glacial conditions prior to abandonment.
Age of the Little Glacier Lake moraines indicate conditions within the Upper Valley had become favorable for glacier activity by 2250±60 to 720±50 years ago with glaciation likely occurring prior to this time (Fig. 6a). These data also imply that subsequent glaciation (e.g., LIA) did not exceed this boundary, which therefore represents the maximum late Holocene glacial extent. Based on geomorphic evidence and variability among exposure ages, we suggest that the Little Glacier Lake moraines represent rock glaciation and formed through transitional glacial and periglacial processes (Petersen et al., 2020). Therefore, continued and variable glacial–periglacial conditions for the Little Glacier Lake rock glacier are demonstrated by the full range of exposure ages until abandonment by 720±50 years at the latest.
In nearby Glacier National Park, MT (∼500 km north of the Beartooth Mountains) proglacial lake sediments are interpreted to indicate glacier fluctuations throughout the Holocene, including phases of glacier advance between 2300 and 1500 cal years BP and again after 700 cal years BP (Fig. 6; Munroe et al., 2012). Paleo-proxy reconstructions of temperature and precipitation for the region indicate lower than average temperatures and increased winter precipitation ∼ 1600–1200 years ago, with continued decreased temperatures ∼700 years ago (Trouet et al., 2013; Viau et al., 2012). Together, these various proxies of climate and glacier activity suggest climate conditions favorable for glaciation marked by small-scale glacier fluctuations and regional variability.
Continuation of climate and glacier variability recorded in ages of the Little Glacier Lake deposits and regional paleo-proxies culminated with the glaciation recorded in the Triangle Lake moraine. Morainal abandonment of the Triangle Lake glacier between 390±20 and 120±10 years ago marks the end of substantial moraine construction within the Upper Valley. Similarities between the Triangle Lake and the up-valley Frosty Lake moraine morphologies imply similar ages and behaviors for the two glaciers. Both moraines are large (>50 m relief), likely from either the long duration of moraine construction at the boundary or high depositional rates. We suggest late Holocene reactivation of the Triangle Lake glacier was synchronous with the onset of glaciation recorded in the Little Glacier Lake deposits, as both responded to the same forcing. Unlike at Little Glacier Lake, the Triangle Lake glacier maintained its position and positive mass balance longer, thus constructing a more substantial moraine and staving off rock glaciation, which can occur as a periglacial feature (Giardino and Vitek, 1988; Knight et al., 2019).
A recent study of glaciers in New Zealand highlighted the inverse hemispheric relationship, as those glaciers reached maximum lengths early in the Holocene and have retreated ever since (Dowling et al., 2021). It is likely that glaciers and periglacial features in the higher elevations of the Beartooth Mountains reactivated in response to late Holocene low NH insolation levels, and provide evidence of cooler and more favorable climates in the last half of the Holocene. Though proximal, differences in Little Glacier Lake and Triangle Lake glacier responses to climate forcing could be driven by the influence of higher and more shaded headwalls surrounding the Triangle Lake glacier. Similar topographic scenarios have been shown to influence alpine glacier advances (Barr and Spagnolo, 2015). Taken together, the Little Glacier Lake and Triangle Lake exposure ages suggest reactivation of glaciers within the Upper Valley from their early Holocene minima during the Neoglaciation prior to 2250±60 years, with deglaciation occurring as late as 120±10 years. Exposure ages from the Little Glacier Lake deposits support regional studies suggestive of glacier fluctuations and climate variability throughout the Neoglaciation.
New 10Be surface exposure ages from the Rock Creek drainage in the eastern Beartooth Mountains yield new insight into the timing of western US glaciation. After likely retreating from the LGM limit between 22 and 18 ka, the glacier stabilized in the Upper Valley during the Younger Dryas stadial, as evidenced by the Emerald Lake moraine (12.5±0.7 ka). This age redefines the age of the deposit, which was initially suggested to be Neoglacial in age by Graf (1971), and fits a trend of previously assigned Neoglacial deposits being geochronologically constrained to latest Pleistocene and earliest Holocene glaciation (Marcott et al., 2019). Evidence of Neoglaciation is found within the Upper Valley in the presence of the Little Glacier Lake lateral moraines that yield a range of exposure ages between 2250±60 and 1060±40 years, with continued glacial–periglacial rock glacier fluctuations occurring throughout the late Holocene between 2030±60 and 720±50 years. These deposits support evidence that climatic conditions within the western US were favorable for glaciation at high elevations in the late Holocene (Marcott et al., 2019; Solomina et al., 2016). Glaciation continued within the Upper Valley until retreat of the Triangle Lake glacier between 390±20 and 120±10 years, with only ephemeral glaciers and ice patches remaining today.
Data from this study and those used in figures will be uploaded to the PANGAEA database and available to other researchers in the near future.
AMB, SAM, and JDS conceptualized the project. All authors contributed to the investigation of this project. The original draft and figures were written and created by AMB with reviewing and editing by all other co-authors.
The contact author has declared that none of the authors has any competing interests.
Publisher's note: Copernicus Publications remains neutral with regard to jurisdictional claims in published maps and institutional affiliations.
We thank Cameron Batchelor for their assistance with fieldwork, and the two anonymous reviewers for their helpful comments, which greatly improved this paper. Aaron M. Barth, Elizabeth G. Ceperley, Claire Vavrus, and Shaun A. Marcott acknowledge financial support from the University of Wisconsin – Madison during the completion of this project. Aaron M. Barth acknowledges financial support from Rowan University.
This project was supported from the University of Wisconsin – Madison and Rowan University.
This paper was edited by Hella Wittmann-Oelze and reviewed by two anonymous referees.
Balco, G.: Contributions and unrealized potential contributions of cosmogenic-nuclide exposure dating to glacier chronology, 1990–2010, Quaternary Sci. Rev., 30, 3–27, https://doi.org/10.1016/j.quascirev.2010.11.003, 2011.
Balco, G., Stone, J. O., Lifton, N. A., and Dunai, T. J.: A complete and easily accessible means of calculating surface exposure ages or erosion rates from 10Be and 26Al measurements, Quat. Geochronol., 3, 174–195, https://doi.org/10.1016/j.quageo.2007.12.001, 2008.
Ballard, G. A.: Evidence to suggest catastrophic flooding of Clarks Fork of the Yellowstone River, northeastern Wyoming (M.S.), University of Utah, 1976.
Barr, I. D. and Spagnolo, M.: Glacial cirques as palaeoenvironmental indicators: Their potential and limitations, Earth-Sci. Rev., 151, 48–78, https://doi.org/10.1016/j.earscirev.2015.10.004, 2015.
Barth, A. M., Clark, P. U., Clark, J., Roe, G. H., Marcott, S. A., McCabe, A. M., Caffee, M. W., He, F., Cuzzone, J. K., and Dunlop, P.: Persistent millennial-scale glacier fluctuations in Ireland between 24 ka and 10 ka, Geology, 46, 151–154, https://doi.org/10.1130/G39796.1, 2018.
Barth, A. M., Ceperley, E. G., Vavrus, C., Marcott, S. A., Shakun, J. D., and Caffee, M. W.: Beryllium-10 surface exposure age data for moraines in the Beartooth Mountains, Montana, USA, PANGAEA, last access: 5 December 2022.
Bevan, A.: Summary of the Geology of the Beartooth Mountains, Montana, J. Geol., 31, 441–465, https://doi.org/10.1086/623038, 1923.
Bolch, T.: Climate change and glacier retreat in northern Tien Shan (Kazakhstan/Kyrgyzstan) using remote sensing data, Global Planet. Change, 56, 1–12, https://doi.org/10.1016/j.gloplacha.2006.07.009, 2007.
Braumann, S. M., Schaefer, J. M., Neuhuber, S. M., Reitner, J. M., Lüthgens, C., and Fiebig, M.: Holocene glacier change in the Silvretta Massif (Austrian Alps) constrained by a new 10Be chronology, historical records and modern observations, Quaternary Sci. Rev., 245, 106493, https://doi.org/10.1016/j.quascirev.2020.106493, 2020.
Briner, J. P. and Porter, S. C.: Neoglaciation in the American Cordilleras, in: Encyclopedia of Quaternary Science, Elsevier, 1133–1142, https://doi.org/10.1016/B0-444-52747-8/00137-X, 2018.
Catania, G. A., Stearns, L. A., Sutherland, D. A., Fried, M. J., Bartholomaus, T. C., Morlighem, M., Shroyer, E., and Nash, J.: Geometric Controls on Tidewater Glacier Retreat in Central Western Greenland, J. Geophys. Res.-Earth, 123, 2024–2038, https://doi.org/10.1029/2017JF004499, 2018.
Clark, P. U., Dyke, A. S., Shakun, J. D., Carlson, A. E., Clark, J., Wohlfarth, B., Mitrovica, J. X., Hostetler, S. W., and McCabe, A. M.: The Last Glacial Maximum, Science, 325, 710–714, https://doi.org/10.1126/science.1172873, 2009.
Corbett, L. B., Bierman, P. R., and Rood, D. H.: An approach for optimizing in situ cosmogenic 10Be sample preparation, Quat. Geochronol., 33, 24–34, https://doi.org/10.1016/j.quageo.2016.02.001, 2016.
Davis, P.: Holocene glacier fluctuations in the American Cordillera, Quaternary Sci. Rev., 7, 129–157, https://doi.org/10.1016/0277-3791(88)90003-0, 1988.
Davis, P. T., Menounos, B., and Osborn, G.: Holocene and latest Pleistocene alpine glacier fluctuations: a global perspective, Quaternary Sci. Rev., 28, 2021–2033, https://doi.org/10.1016/j.quascirev.2009.05.020, 2009.
Dowling, L., Eaves, S., Norton, K., Mackintosh, A., Anderson, B., Hidy, A., Lorrey, A., Vargo, L., Ryan, M., and Tims, S.: Local summer insolation and greenhouse gas forcing drove warming and glacier retreat in New Zealand during the Holocene, Quaternary Sci. Rev., 266, 107068. https://doi.org/10.1016/j.quascirev.2021.107068, 2021.
Dunai, T. J.: Cosmogenic Nuclides: Principles, Concepts and Applications in the Earth Surface Sciences, Cambridge University Press, United Kingdom, ISBN: 978-0-521-87380-2, 2010.
Giardino, J. R. and Vitek, J. D.: The significance of rock glaciers in the glacial-periglacial landscape continuum, J. Quaternary Sci., 3, 97–103, https://doi.org/10.1002/jqs.3390030111, 1988.
Gillespie, A. R. and Bierman, P. R.: Precision of terrestrial exposure ages and erosion rates estimated from analysis of cosmogenic isotopes produced in situ, J. Geophys. Res., 100, 24637–24649, https://doi.org/10.1029/95JB02911, 1995.
Gosse, J. C. and Phillips, F. M.: Terrestrial in situ cosmogenic nuclides: theory and application, Quaternary Sci. Rev., 20, 1475–1560, 2001.
Gosse, J. C., Evenson, E. B., Klein, J., Lawn, B., and Middleton, R.: Precise cosmogenic 10Be measurements in western North America: Support for a global Younger Dryas cooling event, Geology, 23, 877–880, 1995.
Graf, W. L.: Quantitative Analysis of Pinedale Landforms, Beartooth Mountains, Montana and Wyoming, Arctic Alpine Res., 3, 253–261, https://doi.org/10.2307/1550197, 1971.
Hugonnet, R., McNabb, R., Berthier, E., Menounos, B., Nuth, C., Girod, L., Farinotti, D., Huss, M., Dussaillant, I., Brun, F., and Kääb, A.: Accelerated global glacier mass loss in the early twenty-first century, Nature, 592, 726–731, https://doi.org/10.1038/s41586-021-03436-z, 2021.
Knight, J., Harrison, S., and Jones, D. B.: Rock glaciers and the geomorphological evolution of deglacierizing mountains, Geomorphology, 324, 14–24, https://doi.org/10.1016/j.geomorph.2018.09.020, 2019.
Kohl, C. P. and Nishiizumi, K.: Chemical isolation of quartz for measurement of in-situ-produced cosmogenic nuclides, Geochim. Cosmochim. Ac., 56, 3583–3587, 1992.
Laabs, B. J. C., Licciardi, J. M., Leonard, E. M., Munroe, J. S., and Marchetti, D. W.: Updated cosmogenic chronologies of Pleistocene mountain glaciation in the western United States and associated paleoclimate inferences, Quaternary Sci. Rev., 242, 106427, https://doi.org/10.1016/j.quascirev.2020.106427, 2020.
Licciardi, J.: Alpine Glacier and Pluvial Lake Records of Late Pleistocene Climate Variability in the Western United States, Dissertation, Oregon State University, 2000.
Licciardi, J. M. and Pierce, K. L.: Cosmogenic exposure-age chronologies of Pinedale and Bull Lake glaciations in greater Yellowstone and the Teton Range, USA, Quaternary Sci. Rev., 27, 814–831, https://doi.org/10.1016/j.quascirev.2007.12.005, 2008.
Licciardi, J. M. and Pierce, K. L.: History and dynamics of the Greater Yellowstone Glacial System during the last two glaciations, Quaternary Sci. Rev., 200, 1–33, https://doi.org/10.1016/j.quascirev.2018.08.027, 2018.
Lifton, N., Sato, T., and Dunai, T. J.: Scaling in situ cosmogenic nuclide production rates using analytical approximations to atmospheric cosmic-ray fluxes, Earth Planet. Sc. Lett., 386, 149–160, https://doi.org/10.1016/j.epsl.2013.10.052, 2014.
Lifton, N., Caffee, M., Finkel, R., Marrero, S., Nishiizumi, K., Phillips, F. M., Goehring, B., Gosse, J., Stone, J., Schaefer, J., Theriault, B., Jull, A. J. T., and Fifield, K.: In situ cosmogenic nuclide production rate calibration for the CRONUS-Earth project from Lake Bonneville, Utah, shoreline features, Quat. Geochronol., 26, 56–69, https://doi.org/10.1016/j.quageo.2014.11.002, 2015.
Loomis, S. E., Russell, J. M., Verschuren, D., Morrill, C., De Cort, G., Sinninghe Damsté, J. S., Olago, D., Eggermont, H., Street-Perrott, F. A., and Kelly, M. A.: The tropical lapse rate steepened during the Last Glacial Maximum, Sci. Adv., 3, e1600815, https://doi.org/10.1126/sciadv.1600815, 2017.
Lora, J. M. and Ibarra, D. E.: The North American hydrologic cycle through the last deglaciation, Quaternary Sci. Rev., 226, 105991, https://doi.org/10.1016/j.quascirev.2019.105991, 2019.
Lora, J. M., Mitchell, J. L., Risi, C., and Tripati, A. E.: North Pacific atmospheric rivers and their influence on western North America at the Last Glacial Maximum, Geophys. Res. Lett., 44, 1051–1059, https://doi.org/10.1002/2016GL071541, 2017.
Manabe, S. and Broccoli, A. J.: The influence of continental ice sheets on the climate of an ice age, J. Geophys. Res., 90, 2167–2190, https://doi.org/10.1029/JD090iD01p02167, 1985.
Marcott, S. A.: Late Pleistocene and Holocene Glacier and Climate Change, PhD dissertation, Oregon State University, 281 pp., https://ir.library.oregonstate.edu/concern/graduate_thesis_or_dissertations/3484zm26f (lats access: 5 December 2022), 2011.
Marcott, S. A., Shakun, J. D., Clark, P. U., and Mix, A. C.: A reconstruction of regional and global temperature for the past 11,300 years, Science, 339, 1198–1201, https://doi.org/10.1126/science.1228026, 2013.
Marcott, S. A., Bauska, T. K., Buizert, C., Steig, E. J., Rosen, J. L., Cuffey, K. M., Fudge, T. J., Severinghaus, J. P., Ahn, J., Kalk, M. L., McConnell, J. R., Sowers, T., Taylor, K. C., White, J. W., and Brook, E. J.: Centennial-scale changes in the global carbon cycle during the last deglaciation, Nature, 514, 616–619, https://doi.org/10.1038/nature13799, 2014.
Marcott, S. A., Clark, P. U., Shakun, J. D., Brook, E. J., Davis, P. T., and Caffee, M. W.: 10Be age constraints on latest Pleistocene and Holocene cirque glaciation across the western United States, npj Clim. Atmos. Sci., 2, 5, https://doi.org/10.1038/s41612-019-0062-z. 2019.
McKay, N. P., Kaufman, D. S., Routson, C. C., Erb, M. P., and Zander, P. D.: The Onset and Rate of Holocene Neoglacial Cooling in the Arctic, Geophys. Res. Lett., 45, 12487–12496, https://doi.org/10.1029/2018GL079773, 2018.
Mix, A. C., Lund, D. C., Pisias, N. G., Bodén, P., Bornmalm, L., Lyle, M., and Pike, J.: Rapid climate oscillations in the Northeast Pacific during the last deglaciation reflect Northern and Southern Hemisphere sources, in: Rapid Climate Oscillations in the Northeast Pacific during the Last Deglaciation Reflect Northern and Southern Hemisphere Sources, AGU Monograph, American Geophysical Union, Washington, D.C., 127–148, 1999.
Montgomery, C. W. and Lytwyn, J. N.: Rb-Sr Systematics and Ages of Principal Precambrian Lithologies in the South Snowy Block, Beartooth Mountains, J. Geol., 92, 103–112, https://doi.org/10.1086/628837, 1984.
Munroe, J. S., Crocker, T. A., Giesche, A. M., Rahlson, L. E., Duran, L. T., Bigl, M. F., and Laabs, B. J. C.: A lacustrine-based Neoglacial record for Glacier National Park, Montana, USA, Quaternary Sci. Rev., 53, 39–54, https://doi.org/10.1016/j.quascirev.2012.08.005, 2012.
Nishiizumi, K., Imamura, M., Caffee, M. W., Southon, J. R., Finkel, R. C., and McAninch, J.: Absolute calibration of 10Be AMS standards, Nucl. Instrum. Meth. B, 258, 403–413, https://doi.org/10.1016/j.nimb.2007.01.297, 2007.
Osman, M. B., Tierney, J. E., Zhu, J., Tardif, R., Hakim, G. J., King, J., and Poulsen, C. J.: Globally resolved surface temperatures since the Last Glacial Maximum, Nature, 599, 239–244, https://doi.org/10.1038/s41586-021-03984-4, 2021.
Petersen, E. I., Levy, J. S., Holt, J. W., and Stuurman, C. M.: New insights into ice accumulation at Galena Creek Rock Glacier from radar imaging of its internal structure, J. Glaciol., 66, 1–10, https://doi.org/10.1017/jog.2019.67, 2020.
Porter, S. C. and Denton, G. H.: Chronology of neoglaciation in the North American Cordillera, Am. J. Sci., 265, 177–210, 1967.
Praetorius, S. K., Mix, A. C., Walczak, M. H., Wolhowe, M. D., Addison, J. A., and Prahl, F. G.: North Pacific deglacial hypoxic events linked to abrupt ocean warming, Nature, 527, 362–366, https://doi.org/10.1038/nature15753, 2015.
Praetorius, S. K., Condron, A., Mix, A. C., Walczak, M. H., McKay, J. L., and Du, J.: The role of Northeast Pacific meltwater events in deglacial climate change, Sci. Adv., 6, eaay2915, https://doi.org/10.1126/sciadv.aay2915, 2020.
Putkonen, J., Connolly, J., and Orloff, T.: Landscape evolution degrades the geologic signature of past glaciations, Geomorphology, 97, 208–217, https://doi.org/10.1016/j.geomorph.2007.02.043, 2008.
Reyes, A. V., Wiles, G. C., Smith, D. J., Barclay, D. J., Allen, S., Jackson, S., Larocque, S., Laxton, S., Lewis, D., Calkin, P. E., and Clague, J. J.: Expansion of alpine glaciers in Pacific North America in the first millennium A.D., Geology, 34, 57–60, https://doi.org/10.1130/G21902.1, 2006.
Shakun, J. D., Clark, P. U., He, F., Lifton, N. A., Liu, Z., and Otto-Bliesner, B. L.: Regional and global forcing of glacier retreat during the last deglaciation, Nat. Commun., 6, 8059, https://doi.org/10.1038/ncomms9059, 2015.
Solomina, O. N., Bradley, R. S., Hodgson, D. A., Ivy-Ochs, S., Jomelli, V., Mackintosh, A. N., Nesje, A., Owen, L. A., Wanner, H., Wiles, G. C., and Young, N. E.: Holocene glacier fluctuations, Quaternary Sci. Rev., 111, 9–34, https://doi.org/10.1016/j.quascirev.2014.11.018, 2015.
Solomina, O. N., Bradley, R. S., Jomelli, V., Geirsdottir, A., Kaufman, D. S., Koch, J., McKay, N. P., Masiokas, M., Miller, G., Nesje, A., Nicolussi, K., Owen, L. A., Putnam, A. E., Wanner, H., Wiles, G., and Yang, B.: Glacier fluctuations during the past 2000 years, Quaternary Sci. Rev., 149, 61–90, https://doi.org/10.1016/j.quascirev.2016.04.008, 2016.
Sortor, R. N.: Glacial chronologies of western North American mountain ranges from cosmogenic nuclide dating, PhD dissertation, Tulane University, 2022.
Trouet, V., Diaz, H. F., Wahl, E. R., Viau, A. E., Graham, R., Graham, N., and Cook, E. R.: A 1500-year reconstruction of annual mean temperature for temperate North America on decadal-to-multidecadal time scales, Environ. Res. Lett., 8, 024008, https://doi.org/10.1088/1748-9326/8/2/024008, 2013.
Vacco, D. A., Clark, P. U., Mix, A. C., Cheng, H., and Edwards, R. L.: A speleothem record of Younger Dryas cooling, Klamath Mountains, Oregon, USA, Quaternary Res., 64, 249–256, 2005.
Van Gosen, B. S., Elliot, J. E., LaRock, E. J., du Bray, E. A., Carlson, R. R., and Zientek, M. L.: Generalized geologic map of the Absaroka-Beartooth study area, south-central Montana, Miscellaneous Field Studies Map 2338, https://doi.org/10.3133/mf2338, 2000.
Viau, A. E., Ladd, M., and Gajewski, K.: The climate of North America during the past 2000 years reconstructed from pollen data, Global Planet. Change, 84–85, 75–83, https://doi.org/10.1016/j.gloplacha.2011.09.010, 2012.
Young, N. E., Briner, J. P., Leonard, E. M., Licciardi, J. M., and Lee, K.: Assessing climatic and nonclimatic forcing of Pinedale glaciation and deglaciation in the western United States, Geology, 39, 171–174, https://doi.org/10.1130/g31527.1, 2011.
Zhang, E., Chang, J., Shulmeister, J., Langdon, P., Sun, W., Cao, Y., Yang, X., and Shen, J.: Summer temperature fluctuations in Southwestern China during the end of the LGM and the last deglaciation, Earth Planet. Sc. Lett., 509, 78–87, https://doi.org/10.1016/j.epsl.2018.12.024, 2019.